4.1 Background and development
The cardiovascular system of the mouse follows the standard pattern for most mammalian species and comprises the heart, blood vessels and lymphatics. The heart in all mammalian species has striking similarities and given the extensive use of mice to model cardiac disease phenotypes it is helpful that the mouse heart is very similar morphologically to the human heart, with the exception of the arrangement of some of the venous vessels entering the atria (Wessels and Sedmera 2003). The heart is the first organ to develop in the embryo and is formed from cardiomyocytes and endothelial cells originating from cardiogenic mesoderm. The cardiomyocytes and endothelium initially form tubes which loop and eventually divide into the four chambers (Kaufman and Bard 1999; Savolainen et al. 2009). Additional cell types are incorporated into the final heart structure and include differentiation of epicardium, aortic arches and valves from neural crest cells, coronary arteries from mesenchyme and atrial septum from mesocardium.
4.2 Sampling techniques and morphometry
Mouse heart weights vary between strains (Roth et al. 2002; Sugiyama et al. 2002; Krinke 2004) and although data are available in the literature comparison to concurrent controls (or reference data from the same laboratory) is preferred when investigating experimental variations. Absolute weights are rarely helpful and although comparison of organ to body weight is frequently used, this may not be appropriate where body weight changes are expected. The relationship to brain weight may also not be linear and so other methods of comparison, such as covariance analysis, may be required where subtle changes are suspected (Bailey et al. 2004).
The small size of the mouse heart means that it can be conveniently sectioned longitudinally to show all chambers and valves (Morawietz et al. 2004) (Figure 4.1). While this appears to be a simple technique, in practice the small size may make orientation difficult and it is therefore important to practise and standardize the technique to ensure consistent sampling. It is important to be aware of which anatomical regions are of most interest to ensure these are sampled accurately—for example, the papillary muscle is susceptible to toxicity from a number of drug classes and is easily missed from sections. In some circumstances it may be useful to use the three-section technique (Isaacs 1998), where a cross section is taken from the middle of the ventricles and longitudinal sections of the base and apex of the heart (Figure 4.2). This technique allows more accurate comparison and morphometry of the relative thickness of the interventricular septum and ventricular walls and can be helpful to optimize presentation of the valves. With modern imaging techniques (microCT—Martinez et al. 2009 and echocardiography—Liu and Rigel 2009) detailed morphometric techniques can be made from the heart in vivo. However if these techniques are not available it may be necessary to perform morphometry on fixed sections. If this is the case then the heart should ideally be stopped at a consistent stage of the cardiac cycle to avoid the confounding effects of contraction or relaxation on the apparent thickness of the myocardium during systole and diastole. This is normally achieved by the injection of potassium chloride to stop the heart in diastole.
Figure 4.1 Longitudinal section of mouse heart demonstrating all the cardiac chambers, valves and major vessels at the heart base.

Figure 4.2 Alternative sectioning of heart showing transverse section through ventricles and longitudinal sections of base and apex.

4.3 Artefacts
4.3.1 Perfusion artefacts
Perfusion fixation is commonly used in mice to optimize tissue morphology, particularly in the CNS, and to facilitate subsequent techniques such as immunohistochemistry. The most commonly used perfusion techniques for the CNS involve infusion of fixative directly into the left ventricle or into the ascending aorta via the left ventricle. Perfusion via the left ventricle often leads to artefactual distension of the cardiac chambers particularly if the pressure is too high (Figure 4.3). The distension and subsequent thinning of the ventricular walls, which should not be confused with pathological thinning or atrophy of the ventricular walls such as may be seen in dilated ‘cardiomyopathy’. Other routes for perfusion can be considered and include perfusion via the descending aorta or vena cava for whole animal perfusion, via the descending aorta proximal to the distal bifurcation for the kidney and the portal vein for the liver. Even optimized perfusion also leads to changes in the appearance of routinely stained tissue due to the absence of blood cells within the blood vessels (Figure 4.4). The tissue can look strikingly different from tissue that has not been perfused and this can lead to variation within experiments where some animals are well perfused and others are not. Where the perfusion pressure is too high this can lead to disruption of tissue architecture and artificial separation of tissue structures, which may be easily confused with oedema (Figure 4.5).
Figure 4.3 (a) Transverse section of nonperfused heart demonstrates the thick walls of the left ventricle and the interventricular septum and the thin right ventricular wall. (b) Transverse section demonstrating the artefactual distension of left and right cardiac chambers and thinning of the wall due to intracardiac perfusion at necropsy.

Figure 4.4 (a) Section of kidney from a perfused animal—note the absence of red blood cells in the blood vessels and glomeruli in comparison to (b) showing kidney from an unperfused mouse.

Figure 4.5 The effect of overperfusion is shown in this section of jejunum where there is distension of the blood vessels and separation of the tissues giving a false impression of oedema.

4.3.2 Contraction artefacts
A number of artefacts can be introduced to cardiac muscle during handling at necropsy. Cutting the muscle followed by rapid immersion fixation of reactive or beating cardiac muscle can result in artefactual contraction bands (Figure 4.6), which may mimic contraction band necrosis (Olmesdahl et al. 1979). Crushing, cutting and handling can also result in the formation of scattered irregular groups of hypereosinophilic fibres (Figure 4.7) (Keenan and Vidal 2006). These hypereosinophilic fibres should be differentiated from the earliest signs of cardiomyofibre degeneration. Cardiomyofibre degeneration will occur within a few hours of insult therefore if hypereosinophilic fibres are the only change present they are most likely to represent an artefact rather than a genuine pathology. Immersion of the heart into chilled normal saline or formalin before incision may help to reduce artefacts.
Figure 4.6 Contraction band artefact in cardiac muscle can occur due to handling and fixation at necropsy.

Figure 4.7 Artefactual eosinophilic fibres need to be distinguished from degeneration. True degenerative fibres may show fragmentation and vacuolation as well as eosinophilia and are normally accompanied by an inflammatory response.

4.4 Anatomy and histology of the heart
The heart is surrounded by the pericardial sac, which is lined by mesothelium. In most routine sections only the visceral pericardium, the mesothelial layer lining the outside of the heart, is visible. Normal mesothelial cells are very thin, squamous cells that originate from the mesoderm. They form a thin monolayer over a thin basement membrane, which lines the body cavities and produce a lubricating fluid secretion. If the mesothelial cells are perturbed they tend to become cuboidal. Frond-like projections of fibrous connective tissue with a mesothelial covering can sometimes be found incidentally in the pleura and pericardium.
The epicardium consists of the mesothelial covering and a thin layer of connective tissue immediately below it, which also contains blood vessels and fat, particularly at the heart base. Blood vessels can be seen running between myocardial fibres. The mouse generally has two coronary arteries arising from the left and right aortic sinuses supplying the left and right ventricles respectively. These arteries predominantly occur within the myocardium. The interventricular septum is supplied by septal arteries, which may arise from left or right coronary arteries (Fernández et al. 2008). The actual pattern of coronary arteries can be quite variable and so care should be taken when interpreting possible phenotypic changes (Fernández et al. 2008).
Beneath the epicardium is the main muscle mass of the heart, the myocardium. The endocardial surface of the ventricles is lined by branching longitudinal folds known as the trabeculae carnae. Unlike in man, there is no clear difference in the width of these trabeculae between the left and right ventricles (Wessels and Sedmera 2003). The myocardium consists predominantly of cardiac myocytes, which are cylindrical, branching fibres that are joined end-to-end to adjacent cells by intercalated discs (Figure 4.8). The intercalated discs are composed of adherens junctions and desmosomes, which help to maintain contact between adjacent fibres during contraction (Li and Radice 2010) and gap junctions which allow transmission of impulses between adjacent fibres. Cardiacmyocytes have one or two centrally placed nuclei. Enlarged cardiomyocyte nuclei are common in mouse hearts (Elwell and Mahler 1999), particularly in the papillary muscle (Figure 4.9), and may indicate polyploidy or a response to injury (Keenan and Vidal 2006; Liu et al. 2010). The arrangement of the contractile proteins within the myofibre leads to the striated appearance. The presence (or absence) of striations can be highlighted using special stains, for example phospho tungstic acid haematoxylin (PTAH) (Figure 4.8) and antibodies to cardiac specific proteins like troponin can be used to demonstrate early signs of myocyte damage. Cardiac myocyte fibres are arranged in a spiral pattern around the heart, so elliptical cross sections are most commonly seen, making it hard to measure myofibre length. Cross-section width can also be hard to measure consistently therefore measurements of cross-sectional area may be more reproducible for morphometry (Hort 1971). Use of silver stain techniques such as Gordon and Sweet’s (Bishop and Louden 1997) for reticulin fibres can be useful to delineate the borders of fibres to facilitate image analysis and morphometry (Figure 4.10). Calcification of the myocardium is a relatively common spontaneous finding particularly in certain mouse strains, such as BALB/c (Figure 4.11). It can occur from a young age, is more common in males and usually affects the right ventricle (Frith and Ward 1988; Taylor 2011). Another common spontaneous finding that can be seen in young mice is ‘cardiomyopathy’, which can have a range of presentations from small clusters of inflammatory cells to foci of myofibre necrosis or fibrosis (Taylor 2011). Comparison of the incidence and severity of these changes with concurrent controls or recent historical controls from the same strain maintained in the same facility is important to establish their significance. Atrial thrombosis is also fairly frequently observed in older mice with significant differences between strains (Taylor 2011).
Figure 4.8 PTAH stained cardiac muscle demonstrating the intetcalated discs (pale pink bands between adjoining fibres) and striations.

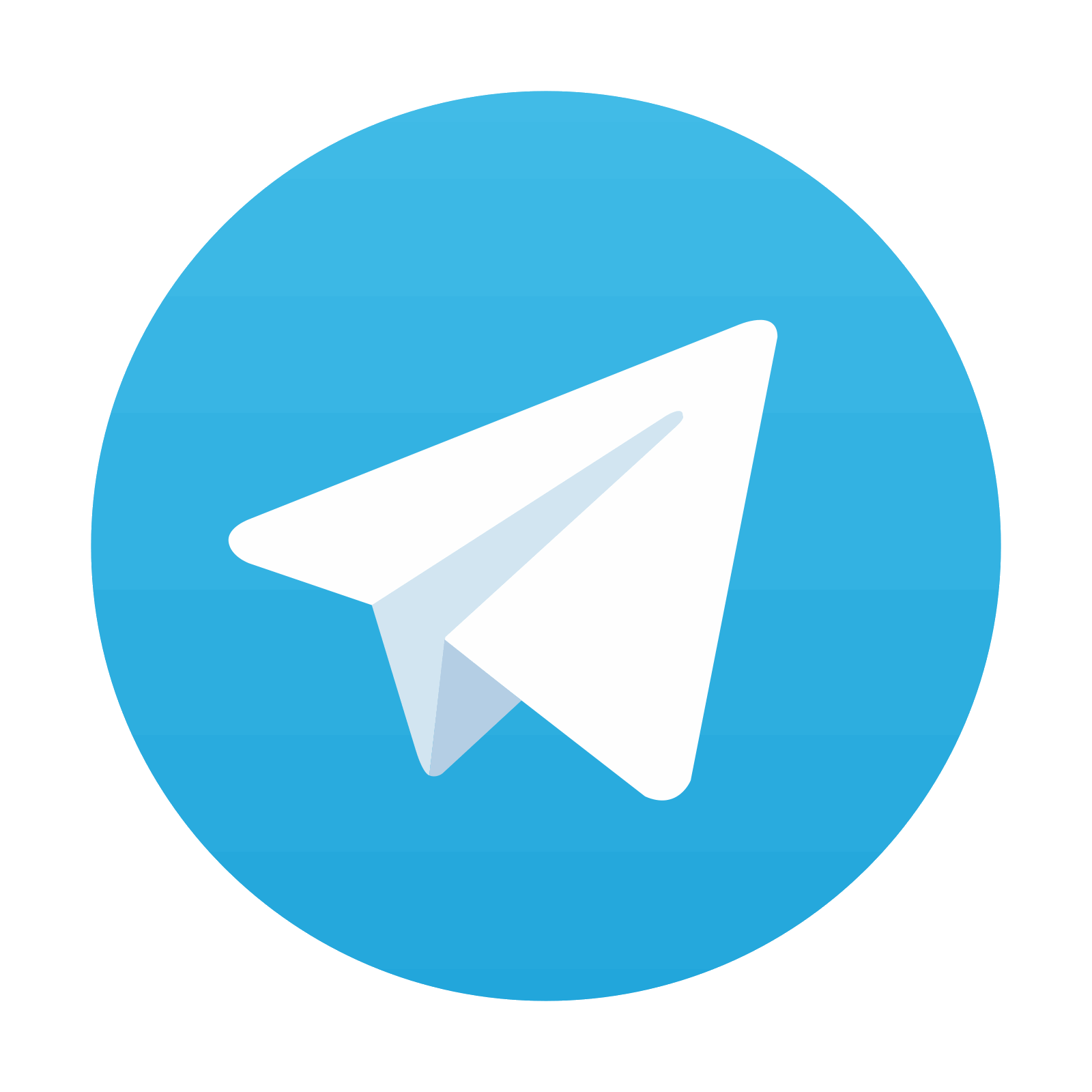
Stay updated, free articles. Join our Telegram channel
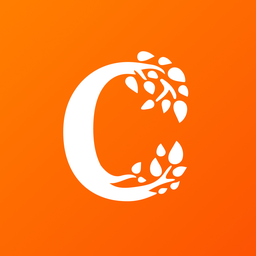
Full access? Get Clinical Tree
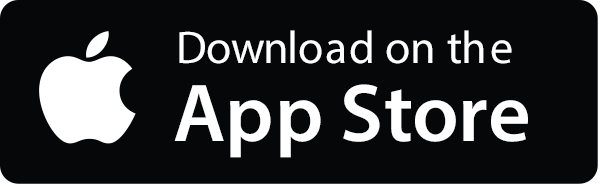
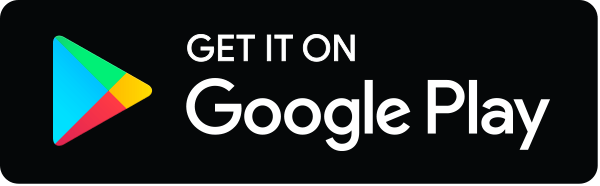