Chapter 3 Behavior and the brain
Chapter contents
Introduction
Only relatively recently has it been accepted that the ‘mental state’ of humans and animals, the emotional, instinctive and cognitive foundations of behavior, are inseparable from their somatic aspects. This artificial schism between psychology and neurology began more than four centuries ago when the philosopher René Descartes was trying to study the human body. The Catholic church expressed its dissatisfaction with this study of God’s handiwork, and in response Descartes struck a deal with the church – human existence was divided into two realms: the physical body, which he would study, and the mental/spiritual realm, which would remain the exclusive domain of the church. This artificial construct dividing the mental and physical has guided much of scientific and medical thought ever since, to the detriment of human and animal patients.1 Increasingly powerful molecular tools are being used by neuroscientists to elucidate the relationship between anatomy, physiology and specific functions in perception, thought and movement. Classic psychiatric diseases are not (yet) clearly defined in horses, but an appreciation of basic neuroanatomy and neurophysiology is fundamental to understanding the influence of neuropathology and psychopharmacology on equine behavior (Fig. 3.1).
Fundamentals of functional and behavioral neuroanatomy
The nervous system processes external stimuli into neuronal impulses resulting in neurotransmitter release, and integrates these with motivations and emotional stimuli to direct the actions of motor units. These enable the animal to react to its environment and influence the behavior of others. The function of the nervous system fundamentally depends on a group of specialized cells called neurons – polarized, elongated cells that are uniquely capable of extremely rapid, intercellular communication. Neurons have a receptor region (the dendritic zone), a cell body (soma) containing the nucleus, and an axon conducting impulses from the dendritic zone to synapse with other neurons (Fig. 3.2). The cell bodies collectively make up the ‘gray’ matter of the central nervous system (CNS), whereas the axons are found in ‘white’ matter. The shape, size and position of the soma as well as the length and branching of the proximal and distal processes differ greatly between neuronal populations. Brainstem axons may have a length of only a few micrometers, whereas the recurrent laryngeal nerve (the nerve that supplies the larynx) is over 3 meters long! Axons often project in groups or bundles that collectively form tracts in the CNS and nerves in the peripheral nervous system. Neurons make up only a small proportion of the total number of cells in the nervous system; the majority are neuroglial cells [Gr. glia, glue] such as astrocytes, cells with important supportive and metabolic functions.
Major components of the central nervous system
This section serves to introduce the fundamentals of neuroanatomy, a subject that has been comprehensively addressed by de Lahunta2 and reviewed by Kaplan & Sadock3 and Behan.4 The CNS consists of the brain and spinal cord (Fig. 3.3). The brain includes the two cerebral hemispheres, which are roughly mirror images of one another, and the brainstem, a narrow structure through which all the pathways entering and leaving the two hemispheres must pass and which comprises the centers that control breathing, heart rate, eye movement and many other critical functions. Caudal to the cerebral hemispheres is the cerebellum, a structure that helps to control movement and balance. The caudal part of the brainstem flows into the spinal cord, the point of exit for nerves on their way out to innervate muscles and the point of entry for sensory fibers returning from the body’s sensory organs. All the nerves outside the central nervous system are collectively called the peripheral nervous system. The two cerebral hemispheres are built around a connecting system of hollow spaces called the ventricular system. The ventricles are filled with cerebrospinal fluid (CSF). This clear fluid also bathes the surface of the CNS, providing mechanical support to the CNS, and playing an important role in maintaining a constant chemical environment. CSF is produced by modified blood vessels known as the choroid plexus located inside the ventricles.
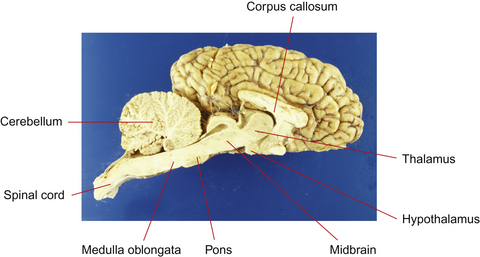
Figure 3.3 Sagittal section of equine brain outlining the major components of the brainstem.
(Photograph courtesy of Keith Ellis.)
There are four major divisions of the CNS:
• forebrain, composed of the cerebral hemispheres, basal nuclei, hypothalamus and thalamus
• brainstem, composed of the midbrain, pons and medulla oblongata
Forebrain
Cerebrum
The cerebrum is the most rostral (forward) division of the brain and is divided into two cerebral hemispheres (Fig. 3.4). Cell bodies of the neurons in the cerebrum are located in two general areas: on the outside surface of the cortex (folded into gyri and sulci) and deep to the surface in the basal nuclei (Figs 3.5 and 3.6). Evidence from human studies suggests that the cerebrum is the region of the brain responsible for perception, emotion, voluntary movement and most learning. These functions will be considered in more detail later.
The tremendous number of neurons in the cerebrum have axons arranged in large fiber bundles. Notably the internal capsule serves as the major projection pathway to connect the cerebrum with the brainstem, and the corpus callosum consists of axons connecting the left and right cerebral hemispheres across the midline. Only one cranial nerve (Table 3.1) – the olfactory nerve, which transmits sensations of smell – is associated with the forebrain (this really is not a ‘nerve’ at all; in the horse it is a relatively large structure, and it probably received its name on account of its diminutive nerve-like appearance in the human brain).
Thalamus and hypothalamus (diencephalon)
The most prominent components of the diencephalon are the thalamus and the hypothalamus. The thalamus functions as an integrating system to relay pathways to and from the brainstem and higher centers in the cerebrum. With the exception of impulses from the olfactory nerve, all impulses heading to the cerebrum must synapse in the thalamus; reciprocal fibers there play a critical role in the filtering of sensory input, and in abnormal states they may generate false signals or inappropriately suppress sensation. The thalamus may also serve directly as the site of conscious perception of some sensations5 and plays a pivotal role in maintaining consciousness, focusing attention, and initiating sleep.6
The hypothalamus is the higher center for regulation of autonomic motor activity. It functions without direct voluntary control, but is influenced by the cerebral cortex. There is evidence that stimuli associated with emotionally relevant events may have a significant effect on hypothalamic hormonal regulation. These hormonal consequences seem to influence further behavior and responses in relevant situations, especially aggression and sexual behavior.7 Seasonal polyestrus behavior of mares is initiated by stimulation of the pineal gland by light, either natural or artificial, causing a reduction of melatonin secretion. This in turn allows gonadotropin-releasing hormone (GnRH) to be secreted by the hypothalamus, ultimately resulting in the secretion of estrogen and estrus behavior (the exact connection between decreased melatonin production and increased GnRH concentrations is not yet understood).
Brainstem
Midbrain
The midbrain is the most rostral portion of the brainstem and contains the pathways connecting the brainstem and cerebrum. It contains the red nucleus, an important nucleus containing ‘upper motor neurons’ that initiate the gait of horses (these are neurons in the brain that innervate and control the lower motor neurons in the brainstem or spinal cord). Two of the three nuclei controlling eye movement – cranial nerves (CNs; see Table 3.1) III (oculomotor) and IV (trochlear) – are found in the midbrain. The dorsal components of the midbrain (rostral and caudal colliculi) are linked with reflex functions such as blinking and, in concert with other structures, reacting (shying) to visual or auditory stimuli.
Medulla oblongata
All of the ascending and descending pathways (directional terms that strictly speaking are only correct for upright primates) between the spinal cord and the brain pass through the medulla oblongata, and the nuclei of the last seven cranial nerves (CNs VI–XII) as well as the sensory nuclei involved in proprioception are located there (Fig. 3.7).
Cerebellum
The cerebellum lies dorsal to the pons. It modulates the tone and relative degrees of contraction of opposing muscles needed for smooth motion, by integrating complex inputs from the brainstem, cerebrum and spinal cord. The reason for the ‘jerky’ movement of foals is because the cerebellum is still developing (but is far, far more developed than the neonatal human brain!). Functional imaging studies in humans have shown the cerebellum to be active during mere imagination of motor acts.8 Foals suffering from a degeneration of the cerebellum, ‘cerebellar abiotrophy’, are strikingly uncoordinated, with strong, jerky movements and prominent tremors, particularly of the head, just before initiating a movement (intention tremors).
Spinal cord
The spinal cord is the most caudal part of the central nervous system. It receives and processes sensory information from the viscera, skin, joints, limbs and trunk, and controls movement of the body, parasympathetic outflow to urogenital structures and sympathetic outflow to the entire body. Neural networks in the spinal cord, referred to as ‘central pattern generators’, produce the rhythmic movements of specific gaits such as trotting, pacing or galloping, even when isolated from the brain and sensory inputs. Supraspinal sensory, and neuromodulatory influences, modulated by training, interact with the central pattern generators to shape the final motor output.9 The gray matter containing the neurons has a roughly H-shaped outline in the center of the spinal cord. Motor neurons are found in the ventral horn, while sensory neurons are present in the dorsal horn. Ascending sensory information passes along fiber tracts (funiculi) to sensory nuclei in the brainstem and cerebellum. Upper motor neurons in the brainstem control movement by innervating lower motor neurons in the ventral horn of the gray matter of the spinal cord, resulting in muscle contraction.
Behavioral neuroanatomy
One organizational principle of the nervous system is the use of parallel processing, in which sensory, motor and cognitive functions can be served by more than one pathway.10 Simplistically, the brainstem and thalamic reticular activating system provide arousal and initiate attention; the caudal part of the forebrain integrates perceptions and, at a conscious level, the frontal cortex initiates voluntary movement and executes plans.
The cerebrum
The cerebral cortex is divided into two hemispheres, which have a contralateral relationship with the body – for example impulses originating in the left hemisphere affect the right half of the body. Within the cortex, human studies have suggested a hemispheric dichotomy of emotional representation, with the left hemisphere housing the analytical mind and the right hemisphere appearing to be dominant in functions involving emotion.11 This may well apply to horses to some extent.
The hemispheres are somewhat arbitrarily divided into four lobes (see Fig. 3.4) named for the overlying bones: frontal, occipital, parietal and temporal. There is a rough correspondence between these loosely defined anatomical regions and their function, a relationship that is most obvious in humans but appears to hold reasonably well for horses. The parietal lobe contains primary somatosensory and motor areas, while the occipital lobe is almost exclusively committed to visual processing. The temporal lobe includes the primary auditory cortex in addition to structures involved in memory, including the hippocampus and the amygdala. The temporal and frontal lobes influence emotions, and epilepsy of the temporal lobes can manifest solely as behavior alterations, such as unprovoked aggression and extreme irrational fear, hyposexuality and tail chasing in small animals.12,13 Temporal lobe epilepsy has not been shown to occur in the horse, but one could reasonably expect that it does.
The limbic system
The components of the limbic system are hard to define, as various authors include different structures and areas in their definitions. It is usually agreed that it consists of those structures forming the border (‘limbic’) of the rostral end of the brainstem. This includes subcortical nuclei such as the hypothalamus and amygdaloid complex as well as the hippocampus, [Gr. hippocampos, sea-horse] an ancient, horseshoe shaped, rolled-up gyrus located within the temporal lobes. In amphibians and reptiles the limbic structures are devoted in large measure to processing olfactory input, a role superseded in mammals by important functions in memory, learning and social and emotional behavior.14
Olfaction remains an important sensory system, and olfaction receptor genes are the largest family of genes currently known to exist. Each receptor protein in the nasal mucosa is highly selective and will bind only a select group of odorants. Horses have a large and well-developed olfactory system which plays a role in appetite and food intake, sexual desire, mating, recognition of friends and foes and the accompanying emotional changes associated with these experiences. An associated sensory apparatus is the vomeronasal organ (VNO), an elongated pouch-like structure ventral to the rostral nasal meatus and lined with olfactory receptors. Scent information, particularly from pheromone molecules, is detected in the VNO (assisted by the ‘flehmen’ response; see Ch. 2). Olfactory signals do not synapse directly in the thalamus but project directly to the frontal lobe and the limbic system. The strong association between odors and memory is rooted in this ancient function of the limbic system.
One nucleus in the limbic system, the amygdala, receives fibers from all sensory areas and appears to assign emotional significance to memories as well as mediating the expression of emotions associated with self-preservation, such as fear.14 Surgical removal of the amygdala results in a loss of fear, changes in social behavior and aggressiveness.
Memory
Learning mechanisms and memory are fundamental to how the brain processes information (see Ch. 4). There is no universally agreed model of how memory works, but it is agreed that a memory is a set of encoded neural connections. Encoding can take place in several parts of the brain, and neural connections are likely to be widespread. Genetically determined positional cues probably steer growing fibers toward the general target with which to synapse, but fine-tuning of the pattern of projections is accomplished by activity-dependent mechanisms. Synaptic relationships are constantly being remodeled through increases or decreases in the size and strength of individual synapses, as well as the formation of new synapses and the elimination of unnecessary ones. This plasticity of cortical representation may not only underlie learning but may allow for recovery from brain lesions.
A widely held hypothesis is that learning occurs through ‘long-term potentiation’, and this has recently been confirmed in rat models.15 Long-term potentiation produces changes in synapses that are necessary to acquire and store new information. Synapses become increasingly sensitive so that a constant level of presynaptic stimulation becomes converted into a larger postsynaptic output. Certain forms of long-term potentiation have been shown to depend on the activation of NMDA receptors (see following sections). Long-term potentiation can be demonstrated in vitro by recording electrical potentials of single hippocampal neurons, and can be prevented by applying NMDA receptor antagonists to the preparations.
The three brain regions that appear to be critical to the formation of memories are the medial temporal lobe and certain thalamic and basal forebrain nuclei. Aside from sensory projection areas, specific areas of cerebral cortex can be damaged with little specific change in learned behavior. Thus these neural substrates are not necessarily the locations in which memory representations are stored but are areas thought to be critical to the normal functioning of the system. It is by modifying the synaptic connections between neurons that processors for most brain functions, including emotion, motivation and motor function, are built.16
Two basic time-scales of learning have been identified: short-term memory and long-term memory (some classifications also include an additional two time scales: immediate and intermediate memory). Neural activity related to short-term memory has been observed in many brain areas, but the mechanism by which this activity can outlast a transient sensory stimulus is still unknown. The transfer of short-term memory (which is easily disrupted) to long-term memory (a more stable anatomical or neurochemical change in the nervous system) depends on the hippocampus and related structures in the medial temporal lobe. Damage to the hippocampus results in an animal’s inability to store recent memories but does not interfere with memories already consolidated before damage occurred.
Cognitive psychological studies have suggested that there are two further distinctions within the domain of long-term memory: the conscious recall of information (explicit memory), and the unconscious use of information about motor skills and procedure (implicit memory). Explicit memory relies on a set of structures in the medial temporal lobe, particularly the hippocampus, diencephalon and gyri in the temporal lobe. The learning of motor skills, allowing movements to be made more quickly and accurately with practice, requires constant feedback from the sensory and association areas for completion but, with practice, these become encoded within a number of cortical areas as well as the basal nuclei and the cerebellum.17 The learning of basic strides by foals as well as the complex responses we expect from horses following sensory cues from the rider, require the formation of long-term implicit memory traces.
Neurophysiology and neurochemistry
Electrophysiology
The ease with which an action potential can be initiated in an individual neuron depends on the relative difference in concentration of sodium and potassium ions inside and outside the axon. The difference is maintained by the action of ion pumps and ion channels. Variation in the resulting electrical difference results in neurons having various thresholds, excitability properties and firing patterns. In the resting state ion channels are closed, but they open in response to the binding of a number of specific molecules at the synapse, or secondary to changes in the membrane potential. Excitatory neurotransmitters act to open cation channels and increase the likelihood of the generation of an action potential. Inhibitory neurotransmitters on the other hand, open anion (chloride) channels that reduce the electrical difference between the inside and outside of the axon membrane and decrease the likelihood of the generation of an action potential. The modulation of the Na1/K1 pump is believed to be one of the fundamental mechanisms for learning,18 by manipulating long-term potentiation.
A single transmitter can produce several distinct effects by activating different types of receptors, and there is now considerable evidence that synapses can be modified functionally and anatomically during development by experience and learning. Training (‘schooling’) of laboratory animals has been shown to produce measurable changes in synaptic interactions.15
Neurotransmitters
Neurotransmitters are classically defined as substances that are synthesized in a neuron, released into the synaptic cleft and then exert a defined action on the postsynaptic neuron by modulating cellular excitability. They are absolutely cardinal to the normal function of the nervous system, and it is worth spending a little bit of time reviewing their function. Their physiology and pharmacology have been well reviewed by Kaplan & Sadock3 and Dodman et al.12 Abnormal levels of neuropeptides have unequivocally been shown to be involved in human psychiatric disorders, and it is also clear that the relative amount and location of neurochemicals can all influence ‘abnormal’ behavior. Rarely if ever can a solitary and unambiguous shift in one neurochemical cause these conditions. Instead, changes in the action of several neurotransmitters or their interaction with receptors are necessary.
Three classes of neurotransmitters classically transmit information in the nervous system: biogenic amines, amino acids and neuropeptides (Table 3.2). Recent data have led to the identification of several novel classes of neurotransmitters, including nucleotides, prostaglandins and gases such as nitric oxide. Some neurotransmitters have in addition been shown to influence gene expression. The field of neurochemistry has breached the bounds of the mere study of chemical mediation of nerve impulses and has developed into a broad discipline that overlaps neuroanatomy, developmental neurobiology and behavioral genetics.
Table 3.2 Classification of neurotransmittersa
Neurotransmitter class | Components | Neurotransmitter |
---|---|---|
Biogenic amines | Quaternary amines | Acetylcholine, histamine |
Catecholamines | Adrenaline (epinephrine) | |
Noradrenaline (norepinephrine) | ||
Dopamine | ||
Indolamines | Serotonin, melatonin | |
Amino acids | Excitatory | Glutamate |
Inhibitory | GABA | |
Inhibitory and excitatory | Glycine | |
Neuropeptides | Calcitonin gene-related peptide | |
Substance P | ||
Vasoactive intestinal peptide | ||
Opioids | Endorphins, enkephalins | |
Hypocretin | ||
Many others | ||
Nucleotides | Adenosine | |
Prostaglandins | Arachidonic acid | |
Gases | Nitric oxide | |
Carbon monoxide |
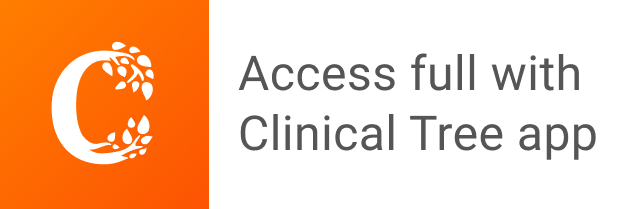