38 Jennifer L. Davis and Lara Maxwell The need for safe and effective antifungal drugs has become important, particularly in small-animal medicine, with the recognition of serious systemic fungal diseases as well as the need for effective drugs to treat skin infections caused by dermatophytes and yeasts. Some animals are at a greater risk of fungal infections because they have other diseases or received medications that can produce immunosuppression, including hyperadrenocorticism, cancer chemotherapeutics, radiation therapy, or prolonged courses of corticosteroids. Fortunately, there have been many advances in the development of antifungal drugs in the last 20 years. Effective oral drugs are more widely used and there are newer, safer formulations of injectable agents. Figure 38.1 illustrates the sites of drug action for common antifungal drugs used in veterinary medicine. Unfortunately, there are only a few antifungal drugs that are approved for veterinary species (notable exceptions are topical products). Therefore, veterinarians often administer human-label drugs in an extralabel manner to animals. Figure 38.1 Schematic anatomy of a fungal cell and potential sites where antifungal drugs act. Griseofulvin (Fulvicin U/F®, Fulvicin P/G®, Grifulvin V®, Grisactin®, Grisactin ultra®), is a fungistatic antibiotic produced by Penicillium griseofulvin dierckx. It is colorless, slightly bitter, and virtually insoluble in water. There are two types of preparations, the microsized and the ultramicrosized. Due to increased surface area, the ultramicrosized formulations have almost 100% bioavailability, whereas oral absorption of microsized formulations is lower and more variable (25–70%). The ultramicrosized preparations are not used often in veterinary medicine because of the higher cost. If the ultramicrosized form is used, the dose must be decreased to account for differences in absorption. Griseofulvin’s selective toxicity is based on an energy-dependent uptake into susceptible fungi that occurs preferentially in fungal cells rather than mammalian cells. Once inside the cell, griseofulvin disrupts the mitotic spindle by interacting with polymerized microtubules, thus causing mitotic arrest in metaphase. Grossly this may appear as shortened fungal hyphae that have fewer branching points. This is known as the curling phenomenon. Griseofulvin may also interfere with cytoplasmic tubule formation, thereby inhibiting normal cellular trafficking. Griseofulvin’s activity is limited to organisms causing dermatophytosis, Microsporum spp., Trichophyton spp., and Epidermophyton. Fungal resistance to griseofulvin, caused by decreased energy-dependent uptake into the fungal cell, has not been reported to be a clinically important problem in veterinary medicine until recently. A 2013 study of feline and canine dermatophytosis showed therapy with griseofulvin failed to achieve both mycological and clinical cure in 16 dogs (39%) and four cats (40%), with some M. gypseum isolates from these animals reaching MIC values of >150 μg/ml (Nardoni et al., 2013). Pharmacokinetic properties were reviewed by Hill et al. (1995). Griseofulvin distributes to the keratin of skin, hair, and nails and can be detected in the stratum corneum within hours of administration. Because of low water solubility, griseofulvin absorption is enhanced when given with a meal with high fat content. Only a small fraction of the dose is present in other body fluids or tissues. Absorption is nonlinear, and increases in doses may lead to a decreased fraction absorbed, as the rate-limiting step for absorption shifts from dissolution to solubility (Tanaka et al., 2013). The plasma half-life in the dog is 47 minutes (Harris and Riegelman, 1969); however, the half-life at the site of action — the stratum corneum — is prolonged because the drug is bound tightly to keratinocytes and remains in the skin until these cells are shed. Thus, new hair or nail growth is first to become free of disease as keratin infected by fungus is replaced by new cells. Griseofulvin is metabolized primarily by the liver to demethylgriseofulvin and the glucuronide. It is metabolized approximately six times faster in animals than in people, which is the reason animal doses are higher than human doses (half-life in dogs is less than 1 hour, compared to 20 hours in people) (Shah et al., 1972). Griseofulvin is still used for treating dermatophytosis, but is being gradually replaced by azole drugs (discussed in Section Azole Antifungal Drugs). The recommended doses have varied, depending on the author. The label dose in dogs and cats for Fulvicin U/F® tablets is 11 to 22 mg/kg/day, but recommendations by specialists in dermatology have ranged from 44 mg/kg/day to 110–132 mg/kg/day in divided treatments (Scott, 1980). One review suggested a dose of 50 mg/kg once a day of the microsize formulation (Hill et al., 1995), and another review listed 25 mg/kg every 12 hours (deJaham et al., 2000), but the dose can be doubled for refractory cases. The most common dose is in the range of 50 mg/kg/day, which was confirmed in a report in which it was used in cats and was as effective as itraconazole for treatment of dermatophytosis (Moriello and DeBoer, 1995). Griseofulvin is available in 125 and 250 mg capsules; 125, 250, and 500 mg tablets; and 125 mg/ml oral syrup. Often, at least 4 weeks are needed for successful therapy, and some patients require 3 months (or more) of continuous therapy. As long as 4 months may be necessary to treat infections of the nail bed (onychomycosis). Griseofulvin is approved at a dose of 2.5 g/day orally in adult horses for a minimum of 10 days. This translates to one packet of the powder formulation or one bolus per day, administered on feed. The dose for foals is half packet or half bolus per foal. Its use is limited to cases of dermatophytosis. There are currently no approved griseofulvin products for use in food animals in the United States. Nevertheless, when used off-label, griseofulvin has been effective in the prevention and treatment of dermatophytes in cattle (Reuss, 1978). Doses used are approximately 7.5–10 mg/kg for 7 to 35 days. At doses of 7.5 mg/kg orally once a day for 7 days, drug metabolites were still detectable in the liver of treated cattle at 10 days following the last administration (Tarbin and Fussell, 2013). A dose rate of 1 g/100 kg has been recommended for pigs for a duration of 30–40 days (Kielstein and Gottschalk, 1970). Because this drug is not approved, veterinarians must determine proper food animal withdrawal times for this drug prior to administration. The most serious adverse effects associated with griseofulvin occur in cats and include leukopenia, anemia, increased hepatic enzyme activity, and neurotoxicosis (Helton et al., 1986). Ataxia in a kitten (Levy, 1991) and bone marrow hypoplasia in an 8-year-old cat (Rottman et al., 1991) have been reported. Prolonged treatment of eight cats with griseofulvin at the high end of the dosage range resulted in no untoward clinical, hematological, or hepatic side effects, suggesting that griseofulvin toxicity may be idiosyncratic (Kunkle and Meyer, 1987). Cats with feline immunodeficiency virus (FIV) appear to be at increased risk for griseofulvin-associated neutropenia (Shelton et al., 1990); however, toxicity has also been reported in FIV-negative cats (Rottman et al., 1991). The mechanism of this increased risk is unknown but may involve griseofulvin-enhanced binding of immune complexes to granulocytic cells in infected cats (Shelton et al., 1991).Griseofulvin should never be administered to pregnant cats. Its teratogenicity has been well-documented (Scott et al., 1975; Gruffydd-Jones and Wright, 1977). The teratogenic effects include cranial and skeletal malformations as well as ocular, intestinal, and cardiac problems (Scott et al., 1975). It has been given to pregnant horses with no apparent ill effect (Hiddleston, 1970); however, this may be dependent on the stage of pregnancy in which the drug was given. One report documented bilateral microphthalmia, brachygnathia superior, and palatocheiloschisis of the drug when given to a mare in the second month of pregnancy (Schutte and Van den Ingh, 1997). The labeled products for horses state not to administer to animals with impaired hepatic function. Amphotericin B (Fungizone®, Abelcet®, Amphotec®, AmBisome®) is a polyene antibiotic with a large macrolide ring with a hydrophobic conjugated double-bond chain and a hydrophilic hydroxylated carbon chain and attached sugar (Figure 38.2) (Mechlinsk et al., 1970). It is a yellowish powder that is insoluble in water and somewhat unstable (Bennett, 1990). There are several formulations of amphotericin B available, including the conventional formulation, which is a micellar complex with the bile salt deoxycholate, and newer formulations that are lipid-based complexes. These are less toxic, but also more expensive (reviewed by Plotnick, 2000). Figure 38.2 Amphotericin B. Amphotericin B lipid complex (Abelcet) is a suspension of amphotericin B complexed with two phospholipids. Amphotericin B cholesteryl sulfate complex (Amphotec, ABCD) is a colloidal dispersion of amphotericin B. The liposomal complex of amphotericin B (AmBisome) is a unilamellar liposomal formulation which, when reconstituted, produces small vesicles of encapsulated amphotericin B. Some investigators have attempted to achieve the benefits of lipid formulations without the added cost by mixing the deoxycholate salt in a 10 or 20% lipid solution (Intralipid). This formulation is stable for up to 3 weeks after mixing (Walker et al., 1998); however, the reports of the benefit of this emulsion versus the conventional formulation are inconsistent. In comparison to the conventional formulation of amphotericin B, lipid formulations can be administered at higher doses to produce greater efficacy with less toxicity (Hiemenz and Walsh, 1996). Decreased toxicity is attributed to a selective transfer of the lipid complex amphotericin B, releasing the drug directly to the fungal cell membrane and sparing the mammalian cell membranes. Reduced drug concentrations in the kidneys and diminished release of inflammatory cytokines from amphotericin lipid complex compared to the conventional formulation may also prevent adverse reactions. The major action of amphotericin B is to bind ergosterol in the fungal plasma cell membrane, making the membrane more permeable and resulting in leakage of cell electrolytes and cell death (Brajtburg et al., 1990). At high concentrations, amphotericin B is thought to cause oxidative damage to the fungal cell (Warnock, 1991) or disruption of fungal cell enzymes. The selective toxicity of amphotericin B is based on its decreased binding to the major cell membrane sterol of mammalian cells (cholesterol) as compared to that of fungal cells (ergosterol). Amphotericin B demonstrates concentration-dependent fungicidal activity. There is also a postfungal effect whereby an antifungal effect persists after drug concentrations have declined. This property allows for intermittent therapy (e.g., every other day in dogs). The growth of strains of most veterinary fungal pathogens is inhibited in vitro at amphotericin B concentrations between 0.05 and 1.0 μg/ml and there is good correlation between the MIC values and clinical response (O’Day et al., 1987). Because of concentration-dependent killing, peak (Cmax) concentrations should be two to four times above the MIC (Cmax/MIC ratio of 2–4 : 1) (Goodwin and Drew, 2008). Susceptible fungi include Histoplasma capsulatum, Cryptococcus neoformans, Coccidioides immitis, Blastomyces dermatitidis, Candida spp., and various species of Aspergillus. Amphotericin B has been indicated for treatment of mucormycosis, sporotrichosis, and phycomycosis (Drouhet and Dupont, 1987). Most strains of Pseudallescheria boydii, as well as some agents causing chromoblastomycosis and phaeohyphomycosis, are resistant to amphotericin. Clinical fungal resistance to amphotericin B, either primary or acquired, does not appear to occur commonly, although resistant strains occur in vitro. In most cases, these resistant strains contain decreased levels of membrane ergosterol (Pierce et al., 1978) and increased catalase levels may allow these fungi to be resistant to oxidative damage (Sokol-Anderson et al., 1988). The MIC concentrations were increased in some human patient populations, such as neutropenic patients (Dick et al., 1980), transplant patients (Powderly et al., 1988), and patients undergoing cytotoxic therapy. The spectrum of activity also includes the protozoa Leishmania and is often included in protocols to treat human and canine leishmaniasis. The treatment of protozoa is discussed in Chapter 42. Despite its long history of use, much is still unknown concerning the pharmacokinetics of amphotericin B, especially in veterinary medicine. It is poorly absorbed from the gastrointestinal (GI) tract and therefore must be given intravenously, locally, or intrathecally. Amphotericin B binds extensively (∼95%) to serum proteins, mainly β-lipoprotein (Bennett, 1977). Much of the drug is thought to leave the vascular space and bind to cholesterol-containing membranes. The highest concentrations are found in liver, spleen, kidney, and lungs, with little accumulation in either muscle or adipose tissue. Concentrations of amphotericin B in fluids from inflamed pleura, peritoneum, synovium, and aqueous humor are about two-thirds of those in serum. Amphotericin B readily crosses the human placenta. Penetration into normal or inflamed meninges, vitreous humor, and normal amniotic fluid is poor. This differential distribution may explain treatment failures for infections in some tissues. Although amphotericin B binds ergosterol with higher affinity than cholesterol, it was suggested that because there are more binding sites for cholesterol in the body than for ergosterol, amphotericin B may be sequestered from its site of action (Bennett, 1977). Amphotericin B is used to treat a variety of fungal diseases caused by susceptible fungi, as listed above. Numerous dosage protocols for amphotericin B have been described in the veterinary literature. These are summarized in Table 38.1. One such protocol for small animals was published by Rubin (1986) that is still used today. During infusion, it should be mixed with 5% dextrose solution because it will precipitate if added to an electrolyte containing solution (e.g., lactated Ringer’s solution). A solution of amphotericin B with 0.45% saline and 2.5% dextrose has been used successfully subcutaneously without any visible precipitation (Malik et al., 1996). Table 38.1 Selected dosing protocols for amphotericin B in companion animals Amphotericin B is used only sporadically as a systemic antifungal in equine medicine and there are no pharmacokinetic data available on amphotericin in the horse. It is more often used as a local treatment in the eye, limbs, and upper airway. In cases of ocular fungal disease that do not respond to typical therapy, amphotericin B (0.2 ml of a 5 mg/ml solution) injected subconjunctivally q 48 h for up to three treatments can be used. This should provide a higher level of drug to the eye; however, it may produce localized toxic effects, including conjunctivitis and conjunctival necrosis. Intravenous regional limb perfusion (IRLP) has also been reported to successfully treat pythiosis of the lower limbs at a dose of 50 mg for one to two treatments (Dória et al., 2012). Side effects include limb edema with pain on palpation, and inflammation of the injection site; however, these signs are considered manageable and resolve after 14 days. Topical and intralesional therapy with amphotericin B has also been reported as a successful treatment for nasal conidiobolomycosis in the horse (French et al., 1985; Zamos et al., 1996). There are no reports of use of this drug in food animals, and there are no approved formulations for use in these species. Combination therapy using amphotericin B and flucytosine has been shown to be synergistic against cryptococcal infections (see Section Flucytosine). Combinations of amphotericin B and azole antifungals have been less successful. Azole-induced depletion of fungal cell membrane ergosterol results in fewer binding sites on which polyene antifungals can exert their effect. Antagonism and synergism between these two classes of antifungal agents have been reported experimentally (Polak et al., 1982; Dupont and Drouhet, 1979). Because of the slower onset of action of azole antifungals, many clinicians recommend initial therapy of serious systemic fungal infections with amphotericin B, followed by longer, follow-up treatment with an azole therapeutic protocol. The most important clinical toxicosis associated with amphotericin B therapy is nephrotoxicity. It is this effect on kidneys that is the most common reason for discontinuing therapy with amphotericin B. It is a dose-related, predictable toxic effect that occurs in almost every animal treated with the conventional formulation. Direct tubular damage occurs because amphotericin B binds to cholesterol in the tubular cells, which results in electrolyte leakage from the cells (primarily K+ loss) and renal tubular acidosis (Bennett, 1990). Induced renal vasoconstriction and impaired acid excretion may also contribute to amphotericin B’s renal toxicity (Greene, 1990). Renal vasoconstriction may be caused by induced increases in the eicosanoid synthesis in renal blood vessels. The tubular damage, along with the renal vasoconstriction, leads to both an acute and a chronic cumulative renal toxicosis. Clinically, the signs of kidney injury are seen as increases in creatinine and blood urea nitrogen (BUN). Electrolyte loading, fluid diuresis, and slow infusion of amphotericin B have all been shown to decrease the severity and the rate of development of renal toxicity. Therefore, common protocols for administration of amphotericin B to animals include pretreatment with sodium chloride IV solution (Rubin, 1986) with or without mannitol (Legendre et al., 1984), and a slow infusion. Slower infusion times are associated with less kidney injury (Rubin, 1986). If the dose administered during a single infusion exceeds 1 mg/kg, acute renal injury is likely (Butler and Hill, 1964). Careful clinical monitoring will help decrease the risk of permanent renal injury. Urine sediment evaluation has been suggested to detect kidney injury earlier than serum biochemical alterations (Greene, 1990); thus, urine should be evaluated for proteinuria, cylinduria, and hematuria, as well as specific gravity. In addition, BUN, creatinine, and electrolyte concentrations should be monitored. Therapy should be temporarily discontinued when active urine sediment is detected or the serum creatinine increases. After stopping therapy, patients may undergo a fluid diuresis to decrease the azotemia. If BUN and creatinine return to near-normal reference values, treatment may be resumed. If azotemia does not improve, one should consider an alternative treatment. Other adverse effects from amphotericin that are frequently observed in animals include phlebitis, fever, nausea, and vomiting. Measures to prevent the nausea and vomiting have included administration of antiemetic drugs such as chlorpromazine, maropitant, or metoclopramide prior to infusion (see Chapter 46). Hypokalemia, bronchospasm, and anemia/hemolysis have frequently been reported in humans and therefore should be monitored for in veterinary patients. The azole antifungal drugs have a high safety profile, a broad spectrum of activity, and are available in topical, oral, and intravenous formulations. There are two main categories of azole antifungal drugs, the imidazoles (clotrimazole, miconazole, ketoconazole) and the triazoles (fluconazole, itraconazole, voriconazole) (Figure 38.3). Clotrimazole and miconazole are discussed in the section on topical therapy. The important physicochemical differences between azole antifungal drugs are summarized in Table 38.2. Table 38.2 Comparison of the physicochemical properties and in vitro activity of commonly used azole antifungal drugs pi, practically insoluble (<0.01 mg/ml); ss, slightly soluble (1–10 mg/ml); vss, very slightly soluble (0–1 mg/ml). Figure 38.3 Azole antifungal drugs All azoles exert their antifungal effect on the cell membrane of fungi by inhibiting synthesis of the primary sterol of the fungal cell membrane, ergosterol. Inhibition of the P450–dependent lanosterol C14-demethylase enzyme results in depletion of ergosterol and accumulation of C14-methyl sterols in the cytoplasmic membrane of yeasts and filamentous fungi. This enzyme is also known as CYP51A or Erg11p and is encoded by the ERG11 gene. Inhibition of this cytochrome P450 enzyme occurs via binding of the nitrogen (N3 of imidazoles and N4 of triazoles) to the heme iron atom of ferric cytochrome P450. This prevents the formation of the superoxide Fe+3 complex (Fe+3O−) needed for hydroxylation of methyl sterols. The result is an inability to demethylate C14-methyl sterols and reduced synthesis of ergosterol. Sterols with less planar configurations are then incorporated into the fungal cell wall, which changes membrane fluidity and interferes with the barrier function of the membrane and with membrane-bound enzymes. Azole drugs are generally fungistatic at concentrations achieved clinically, although there are exceptions for some fungal species, and for some drugs. The parameter that is best associated with clinical cure for azole drugs is the total exposure as measured by the area-under-the-curve in relation to the MIC (AUC/MIC ratio) (Goodwin and Drew, 2008). The potency of each azole drug is related to its affinity for binding the P450 enzyme. The selective toxicity of each compound is directly dependent upon its specificity for binding fungal P450 more readily than mammalian P450. Imidazoles are less specific than triazoles and produce side effects in animals attributed to inhibition of P450 enzymes that are responsible for the synthesis of cortisol and reproductive steroid hormones. Azoles may decrease cholesterol, cortisol, androgen, and testosterone biosynthesis and may interfere with hepatic CYP450 enzymes that are important for drug metabolism and carcinogenic agents (Polak, 1990). These drugs also may inhibit the membrane transporter known as P-glycoprotein. The inhibition of mammalian P450 enzymes is also responsible for drug–drug interactions that have been observed with the azole antifungals. When azoles are administered concurrently with other drugs that are metabolized by these enzymes, they can significantly increase the plasma concentrations of those drugs. Alternatively, when azoles are administered concurrently with drugs that induce the P450 enzymes, the concentrations of the azole drugs may be significantly decreased. The drug–drug interactions important to veterinary medicine are summarized in Table 38.3. The ability to inhibit mammalian P450 enzymes, and therefore the likelihood of drug–drug interactions, is greatest with ketoconazole (Aidasani et al., 2008) followed by itraconazole, voriconazole, and fluconazole. Table 38.3 Antifungal drug–drug interactions of significance in veterinary medicinea aNot all of these interactions have been documented in veterinary medicine, but are present in human medicine and should be monitored for in veterinary patients. Another method by which the azole antifungals can interfere with the absorption and pharmacokinetics of concurrently administered medications is through inhibition of the P-glycoprotein efflux pumps. These efflux pumps can be found in the intestine, where they limit the absorption of some substrates, as well as in the liver, kidney, eye, and CNS. At the intestinal level, there is a relationship between the P-glycoprotein efflux pump and the metabolism by intestinal CYP450 enzymes (Benet, 2009). Inhibition of both can have profound effects on systemic drug concentrations. Azole antifungals have the ability to inhibit P-glycoprotein pumps and therefore increase the oral absorption and tissue distribution of drugs within the body, particularly into protected sites, such as the blood–brain barrier and the blood–retinal barrier. The ability to inhibit P-glycoprotein is greatest with itraconazole, followed by ketoconazole and voriconazole (Wang et al., 2002). Fluconazole has little interaction with P-glycoprotein, which may explain why it has fewer significant drug interactions compared to the other azole antifungals (Yasuda et al., 2002; Wang et al., 2002). Ketoconazole (Nizoral®), one of the imidazoles, became available in 1979. The results of successful use in veterinary medicine were published shortly thereafter (Legendre et al., 1982; Medleau et al., 1985). Ketoconazole is available in 200 mg tablets, and generic formulations are inexpensive and readily available. Ketoconazole is most effective against yeast and dimorphic fungi such as Candida, Malassezia pachydermatis, C. immitis, H. capsulatum, and B. dermatitidis, as well as most dermatophytes with MIC values less than 0.5 μg/ml. It is less effective against C. neoformans, S. schenckii, and Aspergillus spp., with MIC values varying from 6 to >100 μg/ml (Hume and Kerkering, 1983). Ketoconazole is relatively insoluble, except in an acid environment. It is not well absorbed orally unless there is acid secretion, such as after a meal. Ketoconazole is highly protein bound (>98%) and therefore does not penetrate into the cerebrospinal, seminal, or ocular fluid to a significant degree; although it does partition into milk. It distributes throughout the skin and subcutaneous tissue, making it effective for treatment of superficial and systemic fungal skin infections. The drug demonstrates nonlinear absorption and elimination kinetics, most probably due to saturation of solubility or metabolizing enzymes. It is biotransformed in the liver via O-dealkylation and aromatic hydroxylation and excreted mainly in the bile. Elimination half-life is approximately 2 hours in dogs. Because ketoconazole is soluble only in acid aqueous environments (pH <3), gastric alkalizing agents (e.g., antacids, H2 blockers, and parietal cell proton pump inhibitors) or diseases resulting in achlorhydria will decrease its dissolution and oral absorption. Because of lack of consistent gastric acidity, ketoconazole is absorbed poorly in horses. When ketoconazole was administered at 30 mg/kg to horses in corn syrup, the drug was not detected in serum; however, when it was administered with 0.2 N hydrochloric acid intragastrically, oral absorption increased but systemic availability was only 23% with peak serum concentrations of 3.76 μg/ml (Prades et al., 1989). In people, ketoconazole has been replaced in therapy by safer triazole antifungal drugs and is no longer marketed in some countries. But in veterinary medicine, owing to ketoconazole’s efficacy, safety, cost, and ease of administration, it is still a popular antifungal agent. For dermatophytosis in cats, 10 mg/kg/day has been used (Medleau and Chalmers, 1992). For candidiasis, 10 mg/kg/day for 6–8 weeks is recommended. For canine blastomycosis, histoplasmosis, cryptococcosis, and coccidioidomycosis, the dosage is 10–20 mg/kg every 12 hours. Ketoconazole may also be effective in treating nasal cryptococcosis in a dog at a dose of 10 mg/kg/day (Noxon et al., 1986). For Malassezia dermatitis in dogs, dosages of 5–10 mg/kg/day have been recommended (Hill et al., 1995). The duration of treatment is highly variable. Four to six weeks is a minimum for most diseases; many patients with blastomycosis are treated for a minimum of 2 months and as long as 6 months. If there is CNS involvement, particularly with cryptococcosis, higher doses (40 mg/kg) may be necessary to improve penetration into the CNS. Cats have been successfully treated for cryptococcosis with a dosage of 10–15 mg/kg/day (Pentlarge and Martin, 1986; Legendre et al., 1982; Medleau et al., 1985). As complete eradication of the fungal organism is difficult, relapse is common. For this reason, infections should be treated beyond the time clinical signs have resolved. Ketoconazole is not absorbed well orally in horses and it is not recommended. There are no approved formulations for use in food animals. The use of ketoconazole is not limited to the treatment of fungal infections. Because of its inhibitory effect on P450 and P-glycoprotein, administration of ketoconazole concurrently with cyclosporine for the treatment of immune diseases has been used to reduce the dose of cyclosporine by up to 75% and reduce the cost of cyclosporine therapy by 58% (Dahlinger et al., 1998). A study examining blood and skin concentrations of cyclosporine with concurrent administration of ketoconazole at 2.5 mg/kg each showed this regimen to be potentially as effective as cyclosporine alone at 5.0 mg/kg for treatment of canine atopic dermatitis (Gray et al., 2013). Although this may be the most common interaction manipulated for clinical use, other drug–drug interactions have been reported. Ketoconazole (100 mg/day) administered to healthy beagle dogs resulted in down-regulation of intestinal P-glycoprotein and a subsequent increase in the AUC of orally administered prednisolone (Van der Heyden et al., 2012). Concurrent administration of ketoconazole with methadone significantly increased the AUC and plasma concentrations of methadone after oral administration to healthy Greyhound dogs (Kukanich et al., 2011). Other studies have shown that ketoconazole inhibits its own elimination, as well as that of midazolam in healthy Greyhounds, without any significant effect on fentanyl or morphine elimination (Kukanich and Hubin, 2010; Kukanich and Borum, 2008). Colchicine toxicity has also been reported to be precipitated by ketoconazole in a dog, and these drugs are not recommended for coadministration (McAlister et al., 2014). Ketoconazole inhibits the synthesis of steroid hormones (via inhibition of the cytochrome P450 enzymes), most notably cortisol and testosterone. Although this may be a side effect of therapy, it has been exploited for the temporary management of hyperadrenocorticism in dogs (Bruyette and Feldman, 1988; Feldman et al., 1990) and as an antiandrogen treatment. Steroid synthesis inhibition is a temporary effect that persists only during dosing with ketoconazole (e.g., for up to 8 hours). Although the effects are temporary, they are effective. A recent retrospective study showed that ketoconazole administration improved clinical signs of hyperadrenocorticism in 90% of treated dogs, and 69% of dogs had cortisol concentrations following adrenocorticotropic hormone (ACTH) stimulation that were within the normal range (Lien and Huang, 2008). Dogs in that study were treated for the remainder of their life, with a median survival time after diagnosis of 25 months. Ketoconazole will not produce permanent hypoadrenocorticism. Nausea, anorexia, and vomiting are the most common adverse effects, and may require cessation of therapy, particularly in cats (Medleau and Chalmers, 1992). They are usually dose related and may be diminished by decreasing the dose, dividing the total dose into smaller doses, and administering each dose with food. With chronic therapy pruritus, alopecia, lightening and drying of the hair coat, and weight loss may occur (Greene, 1990). Slight to moderate elevations of inducible hepatic enzymes are expected and may not be accompanied by hepatic injury. However, high elevations in hepatic enzymes, accompanied by other parameters (hyperbilirubinemia and clinical signs consistent with hepatic disease), may indicate hepatotoxicosis. Idiosyncratic hepatitis has been reported in animals and people (Janssen and Symoens, 1983). Ketoconazole is a very potent inhibitor of fungal P450, but it also inhibits mammalian P450 at relatively low concentrations (Aidasani et al., 2008); therefore, side effects and drug interactions can occur. Inhibition of P450(17α) catalyzed conversion of progestins to androgens occurs during treatment. Dose-related inhibition of testosterone has resulted in gynecomastia, sexual impotence, and azoospermia. Cats appear to be more sensitive to ketoconazole liver toxicity than are dogs but they are less sensitive to the hormonal suppressive side effects (Willard et al., 1986a, 1986b). Ketoconazole has been shown to be teratogenic in the rat and has resulted in mummified fetuses and stillbirths in dogs. It is therefore not recommended for use in pregnant or lactating animals. Cataracts have been reported after long-term ketoconazole therapy in dogs (de Costa et al., 1996). The average duration of therapy in affected dogs was 15 months, and dosages ranged from 6 to 31 mg/kg/day. These dogs were not diabetic. The mechanism of this reaction is not known. Fluconazole (Diflucan® and generic) has replaced ketoconazole in small animals and birds for many indications. The triazole groups result in increased resistance to metabolic attack, in vivo potencies 100 times that of ketoconazole, and significantly increased aqueous solubility (8 mg/ml) (Richardson et al., 1990). Because of these properties, this compound has good efficacy in animal models and pharmacokinetic properties that are improved over other azole antifungal drugs such as ketoconazole or itraconazole. It is available in 50–200 mg tablets, powder for oral suspension, and a 2 mg/ml parenteral formulation. Compounded formulations have good oral bioavailability and can be used with a reasonable expectation of performance. Fluconazole has been shown to be effective for animal infections caused by Blastomyces, Candida, Coccidioides, Cryptococcus, and Histoplasma. It is not particularly active against Aspergillus. Resistant Aspergillus strains have been increasing in human medicine with MICs often >256 μg/ml. For this reason, fluconazole should not be used as a first choice for the treatment of aspergillosis unless susceptibility has been determined. Efficacy of fluconazole in people has been associated with AUC/MIC ratios as being the best predictor of cures. A ratio above 25 is considered desirable for the best outcome (Goodwin and Drew, 2008). Fluconazole has different solubility characteristics than ketoconazole and itraconazole and is absorbed well regardless of the circumstances. Feeding or formulation (liquid versus tablet) does not affect absorption. Fluconazole demonstrates linear absorption kinetics, with bioavailability greater than 90% in most species (Brammer et al., 1990); thus, oral and IV dosages are identical. Maximum fluconazole concentrations are reached 1–4 hours after an oral dose. Unlike other azole antifungals, fluconazole is not highly protein bound. Humphrey et al. (1985) found plasma protein binding to be between 10 and 12% at concentrations of 0.1 and 1 μg/ml in mice, rats, dogs, and humans. Similar protein binding has also been documented in horses (12.3%) at a concentration of 5 μg/ml. Fluconazole’s low molecular weight, water solubility, and high unbound fraction allow it to be readily distributed throughout the body, including privileged spaces that ordinarily exclude many drugs. Drug concentrations in saliva, sputum, skin, nails, blister fluid, and vaginal tissue and secretions were found to be similar to plasma concentrations. The advantages of fluconazole lie in its ability to produce higher CSF concentrations than ketoconazole or itraconazole; therefore, it may be useful for treating mycotic meningitis (Kowalsky and Dixon, 1991). Fluconazole CSF/plasma or CSF/serum concentration ratios range from 0.49 in horses (Latimer et al., 2001) to 0.88 in cats (Vaden et al., 1997). The drug also penetrates well into the aqueous humor with ratios of aqueous : plasma of 0.37 and 0.79 in the horse and cat, respectively. Fluconazole is eliminated principally by the kidney. A unique feature of fluconazole is that this drug is the only one of the azoles that is water soluble and excreted in the urine in an active form; therefore, it may be one of the few drugs useful for treating fungal cystitis. As can be expected with a renally excreted drug, renal dysfunction affects fluconazole’s elimination such that dose adjustments are necessary. When patients with normal kidney function were compared with those with severe renal insufficiency, fluconazole’s elimination half-life nearly tripled (from 30.1 hours to 84.5 hours) (Dudley, 1990). Reduced dosages as well as extended dosing intervals have been recommended for patients with chronic kidney disease. The disparity between renal fluconazole clearance and creatinine clearance suggests that net tubular reabsorption is responsible for the extended half-life. Half-life was measured to be approximately 14 hours in dogs, 13–25 hours in cats (Vaden et al., 1997; Craig et al., 1994), and 38 hours in horses (Latimer et al., 2001). Steady-state concentrations are achieved in 5–7 days; thus, the manufacturer suggests a two-times loading dose during the first 12–24 hours (Dudley, 1990). The lack of significant hepatic metabolism allows for linear elimination kinetics; that is half-life is independent of dose. Fluconazole is most often used for treatment of dermatophytes. Although not as active against Aspergillus or Penicillium as other azoles, it has also been used to treat canine nasal aspergillosis and penicilliosis. Ten affected dogs were treated with 2.5–5 mg/kg/day fluconazole orally for 8 weeks. Six dogs became free of disease 2–4 weeks after cessation of therapy and remained free of disease for at least 6 months. Serum alkaline phosphatase and alanine transaminase activity remained within normal ranges throughout the treatment period, and adverse side effects were not noted (Sharp, 1991). Doses as high as 10–12 mg/kg/day have also been recommended in dogs. Fluconazole is also at least as effective as ketoconazole for the treatment of dogs with Malassezia dermatitis (Sickafoose et al., 2010). Fluconazole is associated with survival to clinical remission in 75% of dogs with blastomycosis, which was not statistically different than the 90% survival with itraconazole (Mazepa et al., 2011). The cost of fluconazole therapy in that study was approximately one-third that of itraconazole, and both drugs caused a similar incidence of hepatotoxicosis (elevated alanine aminotransferase, ALT). For cats with systemic cryptococcosis, clinical studies have shown a benefit from a dose of 100 mg/cat/day in one or two divided doses. A practical dose is one 50-mg tablet per cat, once a day, or twice daily for refractory cases. Other reported doses are in the range of 2.5–5 mg/kg once a day (Hill et al., 1995). Pharmacokinetic studies support a dose of 50 mg/cat per day for nasal or dermal cryptococcosis (Vaden et al., 1997). The doses for exotic animals are listed in Table 38.4. The half-life can be prolonged in reptiles because of the dependence on renal elimination. Thus, the half-life was 138 hours in turtles when injected SC, which allows for treatment once every 5 days (Mallo et al., 2002). Table 38.4 Selected systemic antifungal drugs used in exotic animal species Oral absorption in horses is reported to be greater than 100% (Latimer et al., 2001). From this cited study a dosing regimen of a loading dose of 14 mg/kg orally, followed by 5 mg/kg q 24 h was derived for horses to produce sufficient concentrations in plasma and tissues. This dose has been successful in treating cryptococcal meningitis and optic neuritis (Hart et al., 2008), and nasal conidiobolomycosis lesions in adult horses (Taintor et al., 2004) as well as disseminated candidiasis in foals (Reilly and Palmer, 1994). Fluconazole has been generally well tolerated, with mild adverse effects being reported in 5–30% of cases. The GI tract was most frequently involved, followed by the CNS and skin. Elevations in hepatic enzymes have been observed, in small animals and horses, sometimes necessitating termination of treatment. Hematological abnormalities, including anemia, leukopenia, neutropenia, and thrombocytopenia, have been reported in people. In subacute toxicity studies in dogs, the highest dose tested (30 mg/kg) caused slight increases in liver weight, hepatic fat, and plasma transaminase activity. Although there is no evidence of mutagenicity or carcinogenicity, its use in pregnant patients is not recommended. However, it has been used successfully with no observed adverse effects on the fetus in horses in the seventh and tenth month of gestation (Taintor et al., 2004). Drug–drug interactions are less frequently reported with fluconazole than other azole antifungals; however, they do occur. Fluconazole has been shown to significantly increase cyclosporine concentrations in both normal and renal transplanted dogs (Katayama et al., 2008, 2010a). Treatment with fluconazole has been shown to significantly prolong anesthesia times in horses following induction regimens that include midazolam (Krein et al., 2014). Compared to ketoconazole, there is little evidence of testosterone or other steroid biosynthesis inhibition (Shaw et al., 1987) in human or animal patients (VanCauteren et al., 1987b). Itraconazole (Sporanox®) was approved for use in the United States in 1992. Of several triazole compounds screened, itraconazole, first synthesized in 1980, was selected for further clinical development due to several criteria: (i) 5–100 times better in vitro and in vivo potency than ketoconazole, (ii) good activity against Aspergillus spp., (iii) activity against meningeal cryptococcosis in animal models, (iv) fewer adverse effects compared to ketoconazole, and (v) favorable pharmacokinetics (Cauwenbergh et al., 1987). Itraconazole is a weak base (pKa = 3.7), is highly lipophilic (logP = 5.66), and is practically insoluble in water. There are several different formulations available. The intravenous formulation is rarely used in veterinary medicine due to expense as well as instability once reconstituted. There are three oral formulations available. The oral capsules were the first formulation marketed and they are still commonly used. They are available in 100 mg dose strength, and consist of drug coated onto small sugar spheres. The capsules require an acid environment for dissolution and therefore absorption is often highly variable. There is also an oral solution approved for use in humans that contains 10 mg/ml of itraconazole complexed with hydroxypropyl-β-cyclodextrin, to increase the solubility. This product has been demonstrated to have higher, less variable absorption in humans, cats, and horses (Willems et al., 2001; Boothe et al., 1997; Davis et al., 2005; Mawby et al., 2016) but is bioequivalent to the capsules in dogs (Hasbach et al., 2017). In cats, the oral solution was absorbed five times higher than the oral capsule (Mawby, Whittemore, and Papich unpublished data). A third oral formulation is licensed for use in cats in the United States (Itrafungol, Elanco). This formulation is also an oral solution (10 mg/ml itraconazole) with similar solubilizing agents and excipients as the human formulation. Itraconazole has been tested both in vitro and in vivo against a wide variety of fungi (for review see Perfect et al., 1986; VanCutsem et al., 1987; VanCutsem, 1990; Cauwenbergh and DeDonker, 1987). It was found to be effective against virtually all medically important fungi, including Microsporum, Trichophyton, Candida, Malassezia, Sporothrix, Pythium, Histoplasma, Aspergillus, Blastomyces, Coccidioides, and Cryptococcus. It has little activity against Fusarium sp. Like other azole antifungal drugs, the AUC/MIC is the best surrogate marker to predict efficacy. However, the most often reported drug concentrations from studies in humans have been the plasma concentrations measured at the lowest point (trough, or Cmin) during multiple dosing. In these studies (Goodwin and Drew, 2008) the trough concentrations greater than 0.5 to 1.0 μg/ml have been associated with clinical success. Absorption is increased by an acid environment and when taken with meals and is less variable than ketoconazole absorption. Bioavailability increases from 40% after fasting to 99.8% when given with a meal (VanCauteren et al., 1987a), except in horses. Due to the low solubility of itraconazole, commercially available formulations include solubility enhancers. Without these specialized formulations, absorption is negligible. Comparison of the oral absorption of Sporanox® capsules, generic and compounded itraconazole capsules showed that, in dogs, the formulations are not bioequivalent (Mawby et al., 2014). Although therapeutic concentrations are reached with the generic formulations, relative bioavailability of the compounded capsules is only approximately 5%. The compounded suspension and capsule had negligible oral absorption in cats (Mawby et al., 2016). This feature of itraconazole also has been demonstrated in other species as well, including birds and horses. Thus, the use of compounded itraconazole formulations is not recommended. Itraconazole is highly (99.8%) protein bound (95% to albumin and 5% to red blood cells) (Heykants et al., 1987); however, due to its lipophilicity and even higher affinity for tissue proteins, it is extensively distributed throughout the body. Tissue to plasma concentration ratios range from 1 : 1 in brain to 8 : 1 in keratin to 25 : 1 in fat stores. Highest tissue levels are seen in the liver and adrenal cortex (Heykants et al., 1987). High tissue binding also produces a very large volume of distribution (Troke et al., 1990; Heykants et al., 1990) and low plasma concentrations. Although it does not reach high concentrations in the CSF compared to fluconazole, itraconazole was found to be effective in treating meningeal cryptococcosis in both mouse and guinea pig models (Perfect et al., 1986). Itraconazole is extensively metabolized, with less than 1% of the active drug and approximately 35% of inactive drug (as more than 10 metabolites) excreted in the urine. The major metabolite, hydroxyitraconazole, has similar antifungal activity to the parent drug, and is often found at concentrations two to three times higher than itraconazole in the plasma in humans (Willems et al., 2001). The metabolite to parent drug ratio is reported to be similar in dogs (Yoo et al., 2002); however, this metabolite has not been found in either cats (Itrafungol®, package insert) or horses (Davis et al., 2005). The predominant route of elimination for itraconazole is in the bile. Because of the increased metabolic stability of the triazole ring versus the imidazole ring (Richardson et al., 1990), itraconazole has a longer half-life (17–25 hours) than ketoconazole (8 hours) in humans. There is disagreement about the elimination rate since the terminal half-life in the dog has been reported to be 8–12 hours (VanCauteren et al., 1987a) and 44–58 hours (Heykants et al., 1987). Differences in study methods, assay sensitivity, and pharmacokinetic analysis may account for this discrepancy. More important than plasma half-life, therapeutically active concentrations are maintained much longer in tissues than in plasma. For example, itraconazole can be detected for 4 days in vaginal epithelium and for 4 weeks in skin and nails after cessation of therapy. These long-lasting tissue concentrations account for the ability to administer this drug intermittently for some fungal infections, as will be discussed below in Section Clinical Use. Itraconazole, like ketoconazole, exhibits nonlinear pharmacokinetics; steady-state concentrations were found to be three times higher after 14 days of therapy than those predicted by a single dose, and the half-life was seen to increase from 24 to 36 hours (Heykants et al., 1990). Pertinent to the pharmacokinetics is the ability of itraconazole to inhibit drug metabolizing enzymes. Itraconazole and its metabolites are cytochrome P450 inhibitors (Templeton et al., 2008). Metabolizing enzymes may be saturated producing nonlinearity in elimination. In addition, repeated dosing may produce a time-dependent decrease in clearance and accumulation (Templeton et al., 2008). Therefore, with repeated dosing, the clearance may decrease and half-life increase. Coadministration of itraconazole and cyclosporine has been shown to result in increased cyclosporine concentrations in cats (Katayama et al., 2010b). Itraconazole is one of the most commonly administered oral antifungal agents for small animals. It has no endocrine effects compared to ketoconazole and is better tolerated. Itraconazole is highly bound in plasma and there is strong binding to keratin producing drug concentrations in skin that persist 2–4 weeks after cessation of drug therapy. It may be excreted into the sebum, increasing the concentrations in skin. This allows for pulse dosing for some diseases. Histoplasma, Cryptococcus, and Blastomyces are highly susceptible; Candida, Aspergillus, and Penicillium are less sensitive. Itraconazole also has been used to treat cutaneous leishmaniasis because the Leishmania organism has ergosterol in high concentrations in its cell wall. Itraconazole is probably better tolerated in cats than ketoconazole. Nevertheless, adverse effects are still possible. Since most adverse effects are dose related, one is advised to lower the dose in animals in which adverse effects are observed. One report indicated that there were dose-related GI effects of anorexia and vomiting in cats from administration of itraconazole (Mancianti et al., 1998). The dosing regimens for cats were reviewed by Moriello (2004). Doses in cats vary from 5–10 mg/kg once a day, orally for at least 56 days, to 10 mg/kg once a day, for 28 days, followed by pulse therapy of 1 week on/1 week off. Lower doses of 1.5 to 3 mg/kg once daily for cycles of 15 days at a time are also used. The most recent regimen to be studied is 100 mg capsule per cat every other day for up to 8 weeks. This regimen yielded average therapeutic trough plasma concentrations (>0.5 μg/ml) within 3 weeks, however two of the ten cats in the study developed reversible adverse effects (Middleton et al., 2016). The availability of a commercial form for cats has helped to define the use in this species. As mentioned previously, itraconazole (Itrafungol®) 10 mg/ml oral solution is registered for use in cats to treat dermatophytosis (not registered in the USA). The treatment schedule consists of once-daily doses of 5 mg/kg for three 1-week cycles. After each week of treatment, it should be followed by a week without treatment (week on/week off schedule). This schedule has been evaluated in cats and maintains drug concentrations in hair during the nontreatment phase (Vlaminck and Engelen, 2004). Itraconazole has been compared to ketoconazole, with each drug administered at doses of 10 mg/kg/day for the treatment of experimentally induced feline disseminated cryptococcosis (Medleau et al., 1990). After 3 months of therapy, the infection had been cleared by both drugs as determined by cryptococcal antigen titers and CSF culture. Three months following therapy all animals remained clinically normal, and titers and CSF cultures remained negative. Although both antifungals brought about resolution of the disease, all cats receiving ketoconazole became anorectic and lost weight, requiring dosage adjustments. This was not seen with itraconazole, and in fact the animals receiving this drug gained weight during the study. Itraconazole has also been used in naturally occurring cryptococcal infections (Medleau, 1990), where an increase in treatment failure was noted in cats that were seropositive for FIV or feline leukemia virus (FeLV). In dogs, the most extensive study has been for treatment of blastomycosis (Legendre et al., 1996). In a study of 112 dogs, 5 mg/kg/day was as effective as 10 mg/kg/day. With a 60-day course of therapy, 54% of dogs were cured. Itraconazole has been used to treat ocular and systemic blastomycosis in dogs. When given 5 mg/kg itraconazole twice a day for 60 days, 76% of eyes with posterior segment disease other than optic neuritis and 18% and 13% of eyes with anterior uveitis or endophthalmitis, respectively, recovered (Brooks et al., 1992). Pulse dosing has also been evaluated in dogs. Itraconazole doses of 5 mg/kg PO q 24 h for 2 consecutive days per week for 3 weeks was found to be as effective as a dose of 5 mg/kg PO q 24 h for 21 consecutive days in the treatment of Malassezia dermatitis and otitis (Pinchbeck et al., 2002). Itraconazole has been successfully used in both the prevention and the treatment of aspergillosis in caged birds. A dose of 20 mg/kg daily for at least 30 days was used to successfully treat five of 12 presumed cases of Aspergillus infections in penguins. This same author suggests its prophylactic use in penguin chicks (Shannon, 1992). A different treatment protocol was recommended for aspergillosis in raptors. Birds are treated with 10 mg/kg twice daily in combination with amphotericin B nebulization three times a day for 20 minutes. Treatment for some cases lasted as long as 6 weeks. These authors also recommend the prophylactic use of itraconazole whenever the clinician expects increased risk for the disease (Forbes et al., 1992). Other antifungal dosing regimens in birds and other exotic animal species are listed in Table 38.4. Itraconazole has been reported to be effective in horses for the treatment of mycotic rhinitis, osteomyelitis, and guttural pouch mycosis (Korenek et al., 1994; Foley and Legendre, 1992; Davis and Legendre, 1994). A pharmacokinetic study showed that the oral solution at a dose of 5 mg/kg q 24 h will produce adequate levels in blood and tissues for successful treatment (Davis et al., 2005). However, the use of the oral liquid will require large volumes — most likely requiring intragastric administration in horses — and the drug is very expensive. The oral capsules have a lower bioavailability and higher doses or more frequent dosing intervals are recommended. There are no reports of the use of this drug in food animals, and there are no approved formulations for these species. Itraconazole is up to 125 times more selective for fungal P450 systems than mammalian liver enzymes in certain in vitro preparations (Vanden Bossche, 1987). It also does not inhibit P450 systems in the testis, adrenal, or liver in vivo (Vanden Bossche et al., 1990). In clinical studies, 100 mg of itraconazole given to humans each day for 30 days had no effect on serum testosterone or cortisol levels (DeCoster et al., 1987). Similarly, there were no changes in testosterone and cortisol concentrations in rats and dogs receiving daily itraconazole for at least 1 month. The biochemical basis for the specificity of itraconazole toward fungal P450 is thought to be dependent upon the hydrophobic nonligand portion of the molecule and its affinity for the apoprotein portion of the cytochrome molecule (Vanden Bossche et al., 1990). The resulting lack of significant inhibition of liver microsomal enzymes results in itraconazole’s inability to affect other drugs’ metabolism. Although the clinical significance is as yet unknown, drugs that can inhibit or stimulate liver degradative enzymes are able to alter the pharmacokinetics of itraconazole. Even though itraconazole is primarily cleared by hepatic metabolism, there appears to be no need for dosage adjustments in patients with liver disease (Heykants et al., 1987). As with ketoconazole, itraconazole’s oral absorption is pH dependent; therefore, dosage adjustments may be necessary when gastric pH is increased. Because itraconazole is better tolerated than ketoconazole, it is used as the drug of choice for long-term treatment. Dogs, cats, and exotic and zoo animals have received this drug for weeks without adverse effects. The capsules have been administered for up to 6 months in horses with no reported adverse effects. Nevertheless, adverse effects are still possible. Since most adverse effects are dose related, one is advised to lower the dose in animals in which adverse effects are observed. According to Legendre (1995) about 10% of dogs receiving recommended doses develop hepatic toxicosis. Liver enzyme elevations may occur in 10–15% of dogs. Itraconazole has been well tolerated by clinically ill cats, although one case of fatal drug-induced hepatitis has been reported (Medleau, 1990). Anorexia may occur as a complication of treatment, especially with high doses and high serum concentrations. It usually develops in the second month of therapy in dogs. In cats there seem to be dose-related GI effects of anorexia and vomiting (Mancianti et al., 1998). Drug-related cutaneous vasculitis has also been reported as a complication of itraconazole therapy in dogs (Nichols et al., 2001). Dogs chronically administered itraconazole (2.5, 10, or 40 mg/kg daily for 3 months) had no significant alterations in mortality rate, behavior, appearance, food consumption, body weight, hematological values, serum and urine chemistry, or gross pathology (VanCauteren et al., 1987b). Subacute toxicity studies in rats revealed increased adrenal gland weight and the accumulation of proteinaceous material in the mononuclear phagocyte system at doses of 40 and 160 mg/kg. Since the mononuclear phagocyte system is responsible for clearing the host of a fungal infection, the clinical importance of this toxic effect is undetermined. Although not teratogenic at 10 mg/kg, maternal toxicity, embryo toxicity, and teratogenicity were observed at 40 and 160 mg/kg in rats (VanCauteren et al., 1987b); therefore, its use in pregnant animals is not recommended.
Antifungal and Antiviral Drugs
Antifungal Drugs
Griseofulvin
Mechanism of Action
Spectrum of Activity
Pharmacokinetics
Clinical Use
Small animals:
Large animals:
Adverse Effects
Amphotericin B
Mechanism of Action
Spectrum of Activity
Pharmacokinetics
Clinical Use
Species
Formulation
Disease treated
Dosing protocol
Reference
Canine
Fungizone
Unspecified
Pretreatment with 0.9% sodium chloride followed by infusion of 0.5 mg/kg in 5% dextrose (D5W) over 4–6 hours IV q48h; a test dose of 0.25 mg/kg is sometimes recommended.
Rubin, 1986
Canine
Abelcet
Blastomycosis
Pretreatment with LRS at 2.5 times maintenance for 30 minutes followed by flushing the line with D5W and infusing 1 mg/kg amphotericin in D5W over 2.5 hours IV followed by LRS at 2.5 times maintenance for an additional 2 hours after treatment. Repeat q48h to a total cumulative dose of 8–12 mg/kg.
Krawiec et al., 1996
Canine
Abelcet
Unspecified
2–3 mg/kg IV 3 times per week diluted in 5% dextrose to a concentration of 1 mg/ml for a total of 9–12 treatments (cumulative dose of 24–27 mg/kg).
Grooters and Taboada, 2003
Canine
AmBisome
Leishmaniasis
3–3.3 mg/kg IV.
Oliva et al., 1995
Canine
Fungizone 40 ml sterile water and 10 ml 10% Intralipid
Leishmaniasis
Pretreatment with 50 ml/kg of 0.9% sodium chloride followed by 10 ml/kg 20% mannitol. Drug mixture infused over 30–60 minutes at incrementally increasing dosing from 1–2.5 mg/kg IV twice a week for a minimum of 8 injections.
Lamothe, 2001
Canine/feline
Fungizone in 0.45% saline with 2.5% dextrose
Cryptococcosis
0.5–0.8 mg/kg SC in 400 ml for cats or 500 ml in dogs given twice a week for a cumulative dose of 8–26 mg/kg.
Malik et al., 1996
Feline
Abelcet
Unspecified
1 mg/kg IV 3 times per week diluted in 5% dextrose to a concentration of 1 mg/ml for a total of 12 treatments (cumulative dose of 12 mg/kg).
Grooters and Taboada, 2003
Equine
Fungizone
Phycomycosis
0.38 gradually increased up to 1.47 mg/kg IV in 1 l 5% dextrose once daily.
McMullan et al., 1977
Equine
Fungizone
Pulmonary histoplasmosis
0.3–0.6 mg/kg IV in 1 l 5% dextrose once a day or every other day.
Cornick, 1990
Equine
Fungizone
Systemic candidiasis
0.1–0.5 mg/kg IV in 1 l 5% dextrose infused over 4–6 hours once daily.
Reilly and Palmer, 1994
Equine
Fungizone
Candida arthritis
0.33–0.89 mg/kg IV in 1 l 5% dextrose once a day or every other day.
Madison et al., 1995
Equine
Fungizone
Cryptococcal pneumonia
0.5 mg/kg IV in 1 l 5% dextrose as a 1-hour infusion once a day.
Begg et al., 2004
Avian
Fungizone
Aspergillosis
1.5 mg/kg q8–12h reconstituted in sterile water and then diluted 1 : 50 with 5% dextrose for 3–7 days.
Tully, 2000
Adverse Effects
Azole Antifungal Drugs
Activity
Drug
Solubility
pH Dependent
LogP
Protein binding
Yeasts
Aspergillus
Fusarium
Ketoconazole
pi
Yes
3.78
>90%
+
±
−
Fluconazole
ss
No
0.54
10–12%
+
−
−
Itraconazole
pi
Yes
5.66
>98%
+
±
−
Voriconazole
vss
No
1.81
32–58%
+
+
±
Mechanism of Action
Interactions with Drug Metabolism
Drug/drug class
Result
Griseofulvin
Anticoagulants/coumarin or inandione derivatives
Griseofulvin is a hepatic enzyme inducer, which may increase the metabolism of these drugs, resulting in decreased anticoagulant effects.
Barbiturates
Impaired absorption and therefore possibly impaired effectiveness of griseofulvin.
Amphotericin B
Bone marrow depressants
Increased risk of anemia or other blood dyscrasia.
Corticosteroids
Exacerbation of hypokalemia, particularly with those drugs that have significant mineralocorticoid activity.
Digoxin
Hypokalemia caused by AmpB increases the potential for digitalis toxicity.
Neuromuscular blocking agents
Hypokalemia caused by AmpB enhances the blockade of nondepolarizing agents.
Diuretics
Potassium depleting diuretics will exacerbate hypokalemia.
Flucytosine
Synergism of AmpB with flucytosine may decrease the dose of AmpB necessary, therefore reducing the nephrotoxicity, however AmpB-induced renal dysfunction may increase 5-FC concentrations, thus increasing the potential for blood dyscrasias.
Azole Antifungals
Drugs that increase gastric pH
Decreases the absorption of those drugs with a pH-dependent solubility (ketoconazole and itraconazole only).
Digoxin
Increased plasma concentrations of digoxin resulting from P450 inhibition may lead to increased digitalis toxicity.
Benzodiazepines
Increased plasma concentrations of benzodiazepines, particularly midazolam, resulting from P450 inhibition may result in potentiation of the sedative effects of these drugs.
Glipizide
Increased plasma concentrations of glipizide resulting from P450 inhibition may cause hypoglycemia.
Second-generation antihistamines
Although identified in people, and not animals, there may be increased plasma concentrations of antihistamines resulting from P450 inhibition, which may result in cardiac arrhythmias, including ventricular tachycardia and torsades de pointes; not seen with fluconazole except at very high doses.
Warfarin
Increased plasma concentrations of warfarin resulting from P450 inhibition may cause increased anticoagulant effects and bleeding.
Cisapride
Although identified in people, and not animals, there may be increased plasma concentrations of cisapride resulting from P450 inhibition, which may result in ventricular arrhythmias, including torsades de pointes.
Cyclosporine
Increased plasma concentrations of cyclosporine resulting from P450/P-gp inhibition may require adjustment of cyclosporine doses; has been used clinically to decrease the cost of cyclosporine treatment.
Quinidine
Increased plasma concentrations of quinidine resulting from P450/P-gp inhibition may lead to increased quinidine toxicity.
Nifedipine
Increased plasma concentrations of nifedipine resulting from P450/P-gp inhibition.
Hydrochlorthiazide
Hydrochlorthiazide decreases the renal elimination of fluconazole, resulting in increases of fluconazole plasma concentrations.
Carbemazapine
Induction of P450 enzymes by carbemazapine may decrease the plasma concentrations of antifungal drugs.
Rifampin
Induction of P450 enzymes by rifampin may decrease the plasma concentrations of antifungal drugs.
Phenytoin
Induction of P450 enzymes by phenytoin may decrease the plasma concentrations of antifungal drugs.
Phenobarbital
Induction of P450 enzymes by phenobarbital may decrease the plasma concentrations of antifungal drugs.
Prednisolone
Down-regulation of intestinal P-glycoprotein results in a subsequent increase in the AUC of orally administered prednisolone.
Methadone
Inhibition of P450 enzymes results in significantly increased AUC and plasma concentrations of methadone after oral administration to healthy Greyhound dogs.
Colchicine
Increased risk of colchicine toxicity when coadministered with azole antifungals.
Other azole antifungals
Ketoconazole inhibits its own elimination, resulting in possible increased plasma concentrations over time.
Flucytosine
Bone marrow depressants
May exacerbate bone marrow toxicities.
Nephrotoxic agents
May decrease flucytosine clearance, increasing the potential for bone marrow toxicity.
Terbinafine
There are no reported drug–drug interactions with terbinafine.
Ketoconazole
Spectrum of Activity
Pharmacokinetics
Clinical Use
Adverse Effects
Drug Interactions
Fluconazole
Spectrum of Activity
Pharmacokinetics
Clinical Use
Small animals:
Exotic animals:
Species
Drug
Disease treated
Dosing protocol
Reference
Passerine and Softbill Birds
Fluconazole
Candidiasis
2–5 mg/kg PO q24h for 7–10 days
Dorrestein, 2000
Griseofulvin
Dermatophytosis
20 mg/kg PO q24h for 4–6 weeks
Itraconazole
Aspergillosis
5–10 mg/kg PO q12–24h in orange juice or 0.1N HCl for 14 days
Ketoconazole
Dermatophytosis
20–30 mg/kg PO q12h for 14–30 days
Miconazole
Candidiasis or cryptococcosis
10–20 mg/kg IM or IV q8–24h
Nystatin
Intestinal candidiasis
100,000 IU/l of drinking water or 200,000 IU/kg soft food for 3–6 weeks
Psittacine Birds
Fluconazole
Candidiasis
2–5 mg/kg PO q24 h for 7–10 days
Tully, 2000
Flucytosine
Aspergillosis
60–150 mg/kg PO q12h in adults; 100–250 mg/kg PO q12h in neonates. Usually given in combination with amphotericin B
Itraconazole
Aspergillosis
5–10 mg/kg q12h for 4–5 weeks; 5 mg/kg q24h in African grays
Ketoconazole
Candidiasis
20–30 mg/kg q12h in orange or pineapple juice, lactulose, or methylcellulose for 14–30 days
Voriconazole
Aspergillosis
12–18 mg/kg oral q12h
Flammer et al., 2008
Miconazole
Candidiasis or cryptococcosis
20 mg/kg IV q8h
Nystatin
Intestinal candidiasis
100,000–300,000 IU/kg PO q8–12h
Raptors
Fluconazole
Mycelial candidiasis, systemic mycosis
5–15 mg/kg PO q12h for 14–60 days
Huckabee, 2000
Gastrointestinal and systemic candidiasis
2–5 mg/kg PO q24h for 7–10 days
Flucytosine
Aspergillosis
120 mg/kg PO q6h; 20–30 mg/kg PO q6h for 60–90 days; 50–75 mg/kg PO q8h in combination with amphotericin B
Candidiasis
250 mg/kg PO q12h
Itraconazole
Aspergillosis
15 mg/kg PO q12h for 4–6 weeks
Ketoconazole
Candidiasis
15 mg/kg PO q12h
Aspergillosis
30–60 mg/kg PO q12h for 14–30 days
Nystatin
Intestinal candidiasis
100,000–300,000 IU/kg PO q8–12h
Pet Fish
Itraconazole
Systemic mycoses
1–5 mg/kg q24h in feed for 1–7 days
Mashima and Lewbart, 2000
Ketoconazole
Systemic mycoses
2.5–10 mg/kg PO, IM or ICe
Ferrets
Amphotericin B
Systemic mycoses
0.4–0.8 mg/kg IV once a week to a total dose of 7–25 mg
Williams, 2000
Griseofulvin
Dermatophytosis
25 mg/kg PO q24h for 3–4 weeks
Ketoconazole
Systemic mycoses
10–30 mg/kg PO q12h–24h
Hedgehogs
Griseofulvin
Dermatophytosis
25–50 mg/kg PO q24h
Lightfoot, 2000
Itraconazole
Systemic mycoses
5–10 mg/kg PO q12–24h
Ketoconazole
Systemic yeast/fungal infections
10 mg/kg PO q24h
Nystatin
Yeast infections
30,000 IU/kg PO q8–24h
Marsupials
Griseofulvin
Dermatophytosis
20 mg/kg PO q24h for 30–60 days
Johnson-Delaney, 2000
Nystatin
Candidiasis
5000 IU/kg q8h for 3 days
Rabbits
Amphotericin B
Systemic mycoses
1 mg/kg IV q24h
Ivey and Morrisey, 2000
Fluconazole
Systemic mycoses
25–43 mg/kg slow IV q12h
Griseofulvin
Dermatophytosis
12.5–25 mg/kg PO q12–24h for 10–42 days
Ketoconazole
Dermatophytosis
10–40 mg/kg PO q24h for 14 days
Rodents
Amphotericin B
Candidiasis
0.43 mg/kg PO or 0.11 mg/kg SC in mice
Adamcak and Otten, 2000
Griseofulvin
Dermatophytosis
25 mg/kg PO q24h for 14–28 days in gerbils, guinea pigs, hamsters, and rats; 14 days in mice; 28–40 days in chinchillas
Itraconazole
Systemic mycoses
5 mg/kg PO q24h in guinea pigs; 50–150 mg/kg q24h in mice; 2.5–10 mg/kg PO q24 in rats
Ketoconazole
Systemic mycoses/candidiasis
10–40 mg/kg PO q24h for 14 days in all species
Amphibians
Amphotericin B
Systemic mycoses
1 mg/kg ICe q24h for 14–28 treatments
Walker and Whitaker, 2000
Fluconazole
Systemic mycoses
60 mg/kg PO q24h for 7 days
Itraconazole
Superficial and systemic mycoses
2–10 mg/kg PO q24h for 14–28 days
Ketoconazole
Systemic mycoses
10–20 mg/kg PO q24h for 14–28 days
Chelonians
Amphotericin B
Aspergillosis
1 mg/kg ICe q24h for 2–4 weeks
Bonner, 2000
Griseofulvin
Dermatophytosis
20–40 mg/kg PO q72h for 5 treatments
Itraconazole
Sceloporus sp.
20–30 mg/kg PO q6–8h in the spiny lizard
Ketoconazole
Systemic mycoses
25 mg/kg PO q24h for 2–4 weeks in turtles; 15 mg/kg PO q24h (27°C) in the gopher tortoise
Nystatin
Enteric fungal infections
100,000 IU/kg PO q24h for 10 days in turtles
Reptiles
Amphotericin B
Systemic mycoses
1 mg/kg IT q24h for 14–28 days
Funk, 2000
Fluconazole Fluconazole
Systemic mycoses
2–5 mg/kg PO q24h for 5–21 days in lizards and snakes; can also mix 100 mg with 20 ml of nystatin and give PO at 0.5–0.6 ml/kg For sea turtles, a loading dose of 21 mg/kg, followed by 10 mg/kg every 5 days, injected SC (Mallo et al., 2002)
Griseofulvin
Dermatophytosis
20–40 mg/kg PO q72h for 5 treatments in snakes
Ketoconazole
Superficial and systemic mycoses
25 mg/kg PO q24h for 3 weeks in snakes
Nystatin
Enteric fungal infections
100,000 IU/kg PO q24h
Large animals:
Adverse Effects
Drug Interactions
Itraconazole
Spectrum of Activity
Pharmacokinetics
Clinical use
Small animals:
Cats:
Dogs:
Large animals:
Adverse Effects
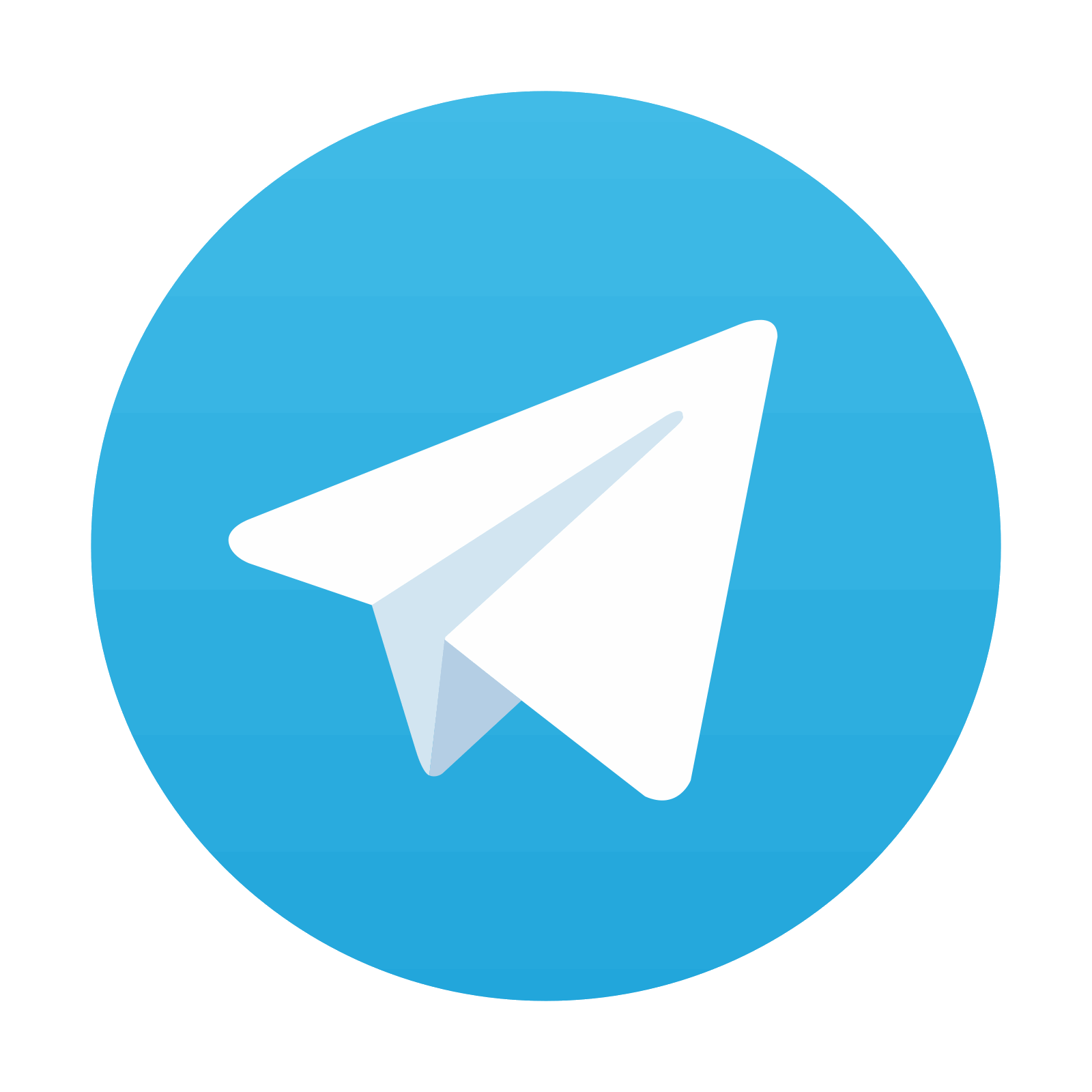
Stay updated, free articles. Join our Telegram channel
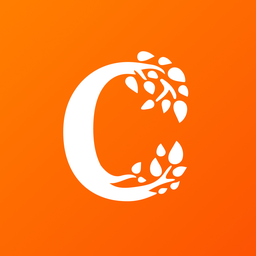
Full access? Get Clinical Tree
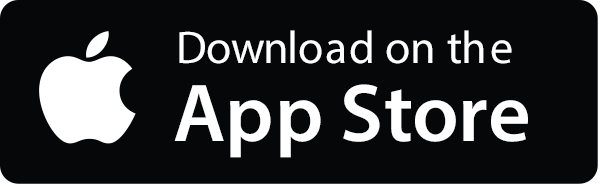
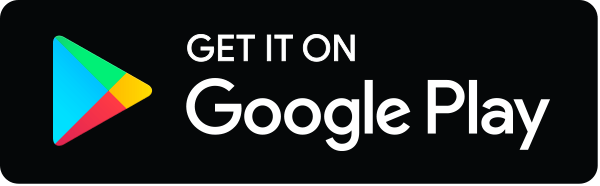