© Springer International Publishing Switzerland 2016
Chi-Chao Chan (ed.)Animal Models of Ophthalmic DiseasesEssentials in Ophthalmology10.1007/978-3-319-19434-9_99. Animal Models of Ocular Tumors
Martine J. Jager1 , Jinfeng Cao1, Hua Yang2, Didier Decaudin3, Helen Kalirai4, Wietske van der Ent5, Nadine E. de Waard1, Nathalie Cassoux6, Mary E. Aronow7, Rohini M. Nair8 and Sarah E. Coupland4
(1)
Department of Ophthalmology, Leiden University Medical Center, Albinusdreef 2, 2333 Leiden, ZA, The Netherlands
(2)
Department of Ophthalmology, School of Medicine, Emory University, Atlanta, Georgia, USA
(3)
Laboratory of Preclinical Investigation, Translational Research Department, Institut Curie, Paris, France
(4)
Pathology, Department of Molecular and Clinical Cancer Medicine, Institute of Translational Research, University of Liverpool, Liverpool, UK
(5)
Institute of Biology, Department of Pathology, Leiden University Medical Center, Leiden University, Leiden, The Netherlands
(6)
Département d’oncologie chirurgicale, Institut and Laboratory of preclinical investigation, Department of Translational Research, Institut Curie, Paris, France
(7)
Clinical Branch, National Eye Institute, National Institutes of Health, Bethesda, MD, USA
(8)
School of Medical Sciences, University of Hyderabad, Hyderabad, India
9.1 Introduction
For many ocular malignancies, surgery, irradiation, or a combination of both are considered the first-line treatments. The success rate of primary therapy is variable among tumor types, but is usually intermediate to high. However, any subsequent local recurrences or metastases usually require specific systemic medications, since either surgery or irradiation may not be possible or effective. The current range of systemic therapeutic agents is limited. In order to broaden the repertoire of cancer-specific targeted therapies, pre-clinical in vitro and in vivo testing is necessary. A wide range of animal models are available, for many different types of malignancies, particularly with the advent of genetic engineering. This chapter will give a summary of the various models used for ocular malignancies, and the different species that can be used.
We will commence with uveal melanoma (UM) and its metastases, followed by conjunctival melanoma , retinoblastoma , and, finally, vitreoretinal lymphoma . Much of the information of this review was obtained from a special edition of “Ocular Oncology and Pathology” (Jager and Coupland, 2015) , in which different animal models are described more extensively: for more details, the readers are referred to the respective articles of this special issue.
9.2 Ocular Models For Uveal Melanoma
UM is a rare malignant tumor , occurring in 4–6 new cases per million people (Singh and Topham 2003) . Tumors may develop in the iris, the ciliary body, or in the choroid, and systemic dissemination occurs mainly hematogeneously. Up to 50 % of patients may develop metastases, which typically occur in the liver. UM has distinct genetic characteristics, which differ from cutaneous melanoma: early mutations are seen in GNAQ or GNA11 (Van Raamsdonk et al. 2009; Van Raamsdonk et al. 2010) . These mutations are mutually exclusive, and experimental work on melanocyte cell lines implanted in mice showed that mutations in these genes play a role in cell division (Van Raamsdonk et al. 2009) . This is comparable to mutations in the BRAF gene in cutaneous melanoma. In order for UM metastases to develop, it is proposed that additional genetic alterations are necessary: these include loss of one copy of chromosome 3 and amplification of chromosome 8q (Sisley et al. 1997; Damato et al. 2010; van den Bosch et al. 2012; Cassoux et al. 2014; Versluis et al. 2015) . One of the most likely candidate genes for metastatic transformation is located on chromosome 3, namely BRCA1 associated protein-1, also termed BAP1: loss of mRNA and protein expression due to bi-allelic gene inactivation is highly correlated with the formation of metastases in UM (Harbour et al. 2010; Shah et al. 2013; Koopmans et al. 2014; van Essen et al. 2014; Kalirai et al. 2014) .
Experience has demonstrated that UM cell lines characterized by chromosome 3 loss and BAP1 deletion are very difficult to culture. Hence, most of the currently available cell lines have disomy 3 and express BAP1 protein (Griewank et al. 2012) . However, recently, BAP1-negative and monosomy 3 cell lines were developed (Amirouchene-Angelozzi et al. 2014) . These differing UM cell lines are now being used to test new therapeutic agents, and to determine whether genetic differences between cell lines influence drug susceptibility. Several drugs display a difference in effectiveness, depending on the presence or absence of GNAQ or GNA11 mutations (Chen et al. 2014; Mitsiades et al. 2011) , and on the chromosome 3/BAP1 status (El Filali 2012) .
Another characteristic of monosomy 3 UM is an inflammatory phenotype (Maat et al. 2008) . Inflammation is recognized as one of the six classic “hallmarks” of cancer (Hanahan and Weinberg 2011) , and may play a role in the initiation of tumor growth and in the formation of metastases. UM contains many macrophages , of which the majority are of the pro-angiogenic M2 type (Bronkhorst et al. 2011) . The special characteristics of the eye as an immune-privileged site allow growth of tumors in the eye, without the need for immunosuppression. This allows, for instance, studies into the role of the innate and adaptive immune system in murine eye tumors (Ly et al. 2010) . However, implantation of human cell lines is often only possible in immunodeficient or immunosuppressed animals.
9.2.1 Spontaneous, Transgenic, and Induced Animal Models
In general, animal models can be essentially divided into three different types: spontaneous, transgenic, and induced models (Cao and Jager 2015) . Examples of spontaneous models are intraocular or conjunctival pigmented lesions that arise in some animals, including cats, dogs, and horses. These tumors develop naturally, but are not good models to be used for experimental therapies, as they occur randomly, are rare, and have an unpredictable and more-often-than-not benign clinical course.
Transgenic models offer a great opportunity to study the early stages of malignancies: particular genes are knocked out or knocked in, and subsequently, tumor growth is induced. Most of the known ocular transgenic UM models to date are murine and induce tumors of the retinal pigment epithelium, possibly with the exception of the Tyr-RAS + Ink4a/Arf−/− transgenic mice (Tolleson et al. 2005) . However, these murine models do not develop metastases, unless UM cells are first cultured and then transferred into them (Ma and Niederkorn 1995) . Non–murine transgenic models include Drosophila and zebrafish. Many biochemical processes are similar in man and Drosophila, and genes can easily be modified to study their effect on cancer processes, for example, the RB1 gene, or genes that play a role in UM, such as the GNAQ and GNA11 genes and the Polycomb complex, which is related to BAP1 (reviewed by Bennett et al. 2015) . The same can be said about the Zebrafish model, which offers particular advantages highlighted in a review by Van der Ent et al. (Van der Ent et al. 2015) .
In induced animal models, tumor development is stimulated by chemical agents, radiation, and xeno-grafted cells or tissues (Cao and Jager 2015). These models are often easier to use for experiments, as they provide reproducible tumors. In UM, the ocular inoculation model is often employed, and tumor cells are injected into the eye, that is, either into the anterior chamber, posterior chamber, or into the subretinal or suprachoroidal spaces. This procedure has been performed on mice, rats, and rabbits (Journee-de Korver et al. 1995; Grossniklaus et al. 1996; Blanco et al. 2000; Rem et al. 2004) . While the rabbit model offers the advantage of a large eye, similar in size to that of the human eye, its main disadvantage is the lack of genetically–inbred strains.
9.2.2 Intraocular UM Models
One of the first UM models was developed in the Syrian gold hamster. A cutaneous melanoma was passaged multiple times and developed into a well-growing amelanotic cell line (Greene Melanoma cell line; Greene 1958) , which grows successfully inside the eye. Journee-de Korver and coworkers (Journee-de Korver et al. 1992; Journee-de Korver et al. 1995) used tissue implants of this tumor to determine the optimal settings to apply thermotherapy; these settings were subsequently used in UM patients treated with transpupillary thermotherapy. Additionally, this cell line was used to test the optimal laser settings for transscleral thermotherapy (Rem et al. 2004). The two problems of the Greene melanoma cell line are its limited availability and its skin melanoma origin. An autologous model does exist in the hamster: the Bomirski cutaneous melanoma will grow when a small piece of tissue is transplanted into the hamster eye (Bomirski et al. 1988; Romanowska-Dixon et al. 2001) . This tumor model continues to be used extensively in Poland.
Significant work has been undertaken to develop rat ocular xenograft models for UM. Braun and coworkers (Braun et al. 2002; Braun and Vistisen 2012) implanted human melanoma cell lines into the eye of athymic albino WAG/RIjHs-mu rats, either by placing a piece of tissue or minced tumor cells into the choroidal space, or by injecting tumor spheroids into the suprachoroidal space. Most spheroid-injected animals developed eye tumors, which can be used to analyze various UM features, for example, angiogenesis, and to test new drugs. A disadvantage of this model is that it does not develop metastases.
As mentioned above, the rabbit eye is larger than the rat eye and has been used as carrier of several melanoma cell lines. The B16F10 cell line is of murine cutaneous origin and tumor fragments obtained from a mouse skin melanoma will grow when placed in the subchoroidal space of the rabbit eye (Krause et al. 2002) . In order for this mouse cell line to successfully grow in the rabbit eye, additional cyclosporin A needs to be administered. In contrast, the Hamster Greene melanoma cell line does not require immunosuppression for growth when placed in a rabbit eye (Romer et al. 1992; Dithmar et al. 2000) . A disadvantage of these tumors, however, is their rapid growth, which limits the time for experiments to 10 days (Schuitmaker et al. 1991) .
Several human cell lines have also been successfully implanted into rabbit eyes, and occasionally metastases have occurred (Blanco et al. 2000) . However, these have tended to spread to the lungs only and did not involve the liver (Blanco et al. 2005) . Lopez-Velasco (Lopez-Velasco et al. 2005) tested four different cell lines and noticed that cell lines 92.1 and SP6.5 grow most aggressively. This model has been used quite successfully (Marshall et al. 2007) .
9.2.3 UM Metastasis Models
The most frequently used induced model for UM is the mouse eye model. The use of ophthalmological instruments and imaging techniques has enabled development of these UM models, despite the small size of the mouse eye. Some of the murine cell lines give rise to metastases, and also some human cell lines can be used to grow metastases in immunosuppressed mice (reviewed by Yang et al. 2015) .
Metastasis models are useful to study the various processes involved in metastasis, that is, tumor cell invasion, migration within the blood stream to the metastatic sites, and the establishment of the metastatic colonies. It is astounding that in human patients, UM metastases can remain dormant for 15–20 years (and in rare cases, even up to 40 years) before becoming clinically manifest, and only then may develop into deadly metastases (Coupland et al. 1996) .
As clinical and genetic data of primary UM can be used to stratify patients into high- and low-metastatic risk groups (Damato and Heimann 2013) , it is essential that new effective therapeutic agents in metastatic UM are identified. However, testing the effect of drugs on the spreading of primary UM is a problem, as very few of the intraocular melanoma models give rise to metastases within a reasonable experimental time limit. To address this, researchers have injected either human or murine UM cell lines directly into the vasculature or into organs of mice, rats, or rabbits, in order to induce tumor spread, for example, intra-arterially, intracardial injections, injections into the spleen or the liver (reviewed by Cao and Jager 2015 and Yang et al. 2015) .
While malignant cells usually grow in the immune-privileged eye, it is unusual for them to disseminate beyond the eye. This is also true for murine and human melanoma cell lines, obtained from either cutaneous or uveal tumors. An exception can be made for UM cell line 92.1 in the rabbit eye (see above), as this tends to provide metastases quickly. Metastases are not easily found in immunosuppressed or immunodeficient mice, not even when using cell lines derived from human UM metastases, such as OMM2.3. One possibility is that the metastases indeed formed, but the mice were not followed for a sufficiently long period, limiting the usefulness of these models. The formation of metastases depends on the interaction between host and cell lines, in which immunological factors such as HLA expression play a role, and on the characteristics of the cell lines themselves. Niederkorn et al. (Ma et al. 1996; Ma and Niederkorn 1998) implanted several human UM cell lines into the anterior chamber of BALB/c athymic mice. Some of them (OCM1 and 92.1) induced liver metastases reproducibly in a large number of mice. It is intriguing that NK cells in the blood of these mice most likely played a role in the difference of metastasis formation, showing the importance of the immune system in tumor cell dissemination. Heegaard (Heegaard et al. 2003) started to implant UM tissues as xenografts subcutaneously in mice. These developed occasionally, but did not lead to metastases. Surriga (Surriga et al. 2013) labeled the OMM1.3 cell line with enhanced green fluorescent protein (EGFP)-luciferase and implanted the cells retro-orbitally in SCID mice. The use of bioluminescent cells should make regular follow-up easier, as otherwise examination for metastases is only possible when the mouse has been killed.
Experimental treatments of targeted therapies can only be tested on human malignancies, carrying the appropriate mutations. UM cell lines have been used for testing medications (de Lange et al. 2012) . Detailed analyses of all available UM cell lines have been undertaken: this is essential to ensure the validity of the cell line as a UM, and to ensure that they have not transformed into another cell line or been inadvertently switched with other cell lines over time (Folberg et al. 2008) . There is a need for the development of increased numbers of UM metastatic cell lines, with characteristic mutational profiles of these tumors.
Studies that will provide more insight into the biology of the eye often use murine cell lines, and some of the murine melanoma cell lines will give rise to visible metastases in syngeneic hosts. B16F10 has been used frequently in syngeneic C57Bl6 mice (Niederkorn 1984; Grossniklaus et al. 1995; Ly et al. 2010; de Lange et al. 2012). This cell line has been used extensively to study UM tumor immunology. A derivative cell line of B16 is the Queens melanoma cell line, which frequently metastasizes, B16LS9, and has been used by Grossniklaus to determine the difference between placing cells in the anterior and posterior chamber: anterior chamber inoculation led to fewer metastases than posterior chamber inoculation (Grossniklaus et al. 1996) . Furthermore, this model has been used to study tumor angiogenesis and tumor immunology. Monoclonal antibodies used against human vascular endothelial growth factor (VEGF) affect blood vessels in this mouse model (Yang et al. 2010) . All these models use murine skin melanoma cell lines in murine eyes.
9.2.4 Human UM Patient-Derived Xenografts
As mentioned above, injecting UM cells retro-orbitally has recently been used to study the effect of a drug, crizotinib (Surriga et al. 2013) . Similar to the experiments of Heegaard, investigators in Paris started to place xenografts derived from primary human UM or from UM metastases in the interscapular fat pad of immunodeficient mice (Garber 2009; Nemati et al. 2010; Decaudin 2011) . Tissue obtained from metastases had a better “take” rate than primary tumor material. These grafts were subsequently used to analyze different drugs, alone or in combination. Panels of UM PDXs with different mutations in GNAQ, GNA11, and BAP1 have significant advantages over the cell line-derived xenografts mentioned above, particularly for testing new drugs with the aim of personalized therapy in UM. These PDXs represent the necessary intermediate step between testing drugs in cell lines and their ultimate application in patients.
In order to create a PDX, tumor fragments are obtained from UM patients and directly implanted into immunodeficient mice. The similarity between the PDX and the patients’ tumor allows the determination of the sensitivity for clinically available drugs or experimental drugs. At the Curie Institute in Paris, samples from 90 UM or their metastases were obtained, and this led to the development of 25 PDXs growing in severe combined immunodeficiency (SCID) mice. The most successful PDXs were those arising from GNA11-mutated UM and their metastases; BAP1 loss or the UM chromosome 3 status did not appear to affect growth (Nemati et al. 2010; Laurent et al. 2013) . The apparent lack of any effect of chromosome 3 may be due to the fact that, of 13 PDXs studied for chromosome status, 10 showed chromosome 3 loss, making further analysis impossible. In vivo passaging did not lead to changes in the main genomic aberrations over time. PDXs showed a complete concordance with the patient material with regard to mutations (GNA11, GNAQ, BAP1, and SF3B1). When looking at the gene expression profiles using an Affymetrix Gene Chip, about 3 % of these genes were found to be differentially expressed between the xenograft and the original UM (Laurent et al. 2013) . The genes that were overexpressed in the patients were associated with the immune system, extracellular matrix, and angiogenic processes, while the genes that were overexpressed in the PDXs were associated with cell cycle, DNA repair, and kinase activity processes. Early and late passages remained the same (Nemati et al. 2010; Carita et al. 2015).
The PDX can be applied for at least two reasons: to screen potential drugs to be used for UM, and to test a drug for a particular patient. It may be possible to initially determine the mutation or genome status of a particular UM, perform an expression array, or determine the presence of specific kinases, and then add the most probable therapeutic agent(s) and study the effect in the mouse PDXs. This model can be applied to test different combinations of drugs. Although this is a very costly procedure to be used in every case, it may ultimately lead to “personalized” medicine in the therapy of metastatic UM, gaining rapid insight into the sensitivity of UM metastases to a specific drug, prior to their ultimate application in patients.
9.2.5 Zebrafish UM Model
While melanoma cells will grow successfully when they are engrafted in mice, tumor cells may also grow in zebrafish (Danio rerio). The embryonic zebrafish can be used as a carrier of UM cells. These cells can be injected into the embryo, and since the adaptive immune system has not yet been developed in the embryo, immunosuppressive drugs are not needed. UM cells can be injected into the yolk sack, the cardiac cavity, or directly into blood vessels, and the ability of the tumor cells to spread can be studied.
The advantages of a zebrafish model include the following: (a) many animals can be obtained and studied simultaneously; (b) noninvasive live imaging is possible due to physical transparency of the developing embryo; and (c) the zebrafish does not have a functional adaptive immune system at early stages of development. This means that immunosuppressive drugs are not required, nor that the experiments are limited to specific immunodeficient strains. Furthermore, the availability of various fluorescent reporter lines aids in investigations by examining the interactions between tumor cells and host. After injection of tumor cells into zebrafish, cell migration, proliferation, and formation of metastases can be studied.
Injection of UM cells into the yolk sack indeed leads to proliferation and migration of UM cells. Injection of biofluorescent cells helps to follow the migration route of the cells and quantify tumor cell burden in vivo. The effect of drugs on all of these processes can be evaluated, and one only has to add the investigational drug to the water in which the fish are maintained. Van der Ent et al. (2014, 2015) tried several injection routes to develop an animal model . Tumor cells that express mCherry were injected into the yolk sac of 2 days post-fertilization (dpf) embryos. Live confocal imaging was performed on embryos at 4, 5, and 6 days post injection (dpi) and the route of migration was analyzed: at 4 dpi, cells started to migrate out of the yolk sac. Migration occurred both to the tail and head region, and also along blood vessels. Cells from the metastasis-derived tumor cell line OMM2.3 showed more migration than of the primary tumor-derived cell lines Mel270 and 92.1. Using this model, it was possible to show the effect of several drugs (Van der Ent et al. 2014, 2015) . Different effects were observed with regard to, for instance, crizotinib, a c-Met inhibitor, which limited migration of c-Met positive OMM2.3 cells, but had hardly any effect on the c-Met low 92.1 cells.
An intriguing finding is that after yolk sac injections, about 10 % of the embryos showed the presence of UM cells in one or both eyes of the embryo (Van der Ent et al. 2015) .
Another route of tumor cell injection is directly into the blood stream of 2 dpf embryos. Cells can be injected into the heart cavity or into the Duct of Cuvier. When using this technique, 80 % of embryos develop intraocular tumor growth. The zebrafish model has been used to grow freshly obtained tumor material as a xenograft (Marques et al. 2009) .
9.2.6 Chick Embryo UM Model
A further way to examine UM metastasis is by utilization of the chick embryo (reviewed by Kalirai et al. 2015) . Tumor cells can be placed on the highly vascularized chorioallantoic membrane (CAM), where a tumor mass will then develop. Tumor cells invade into the CAM’s blood vessels. A second approach is through the injection of tumor cells directly into the blood stream.
The CAM begins to develop by day 4 and by day 12, consists of three layers: the ectoderm, the mesoderm that contains blood vessels, and the endoderm. The ectoderm has a capillary plexus. Additionally, lymph vessels start to develop. The immune system is not yet developed at this time. The chick embryo has been used for xenografting of tumor cells for over a century (Rous and Murphy 1912), but studies on UM are from recent years. Luyten used this model to inject UM cells directly into the eye (Luyten et al. 1993) , where tumor growth was observed.
Kalirai et al. (2015) used the CAM assay: space is made above the CAM inside the egg by removing some albumen. A small opening is made in the shell of the egg, and UM cells can be placed on the CAM through this window. Cell lines OMM1 and 92.1 were engrafted to an area with blood vessels on day 7, and after 1 week, a tumor nodule had developed. These cell lines showed a high Ki-67 proliferation index, Melan-A expression, and vascular structures. Berube (Berube et al. 2005) achieved a similar result with four other UM cell lines.
Green fluorescent protein (GFP)-labeled OMM1 and 92.1 cells were also directly injected into the circulation (Luyten et al. 1996; De Waard-Siebinga et al. 1995; Kalirai et al. 2015) . Cells specifically homed to the neural crest-derived tissues, including the uveal tract of the eye and the liver (Kalirai et al. 2015) . This intravascular model can be used to examine the differences between genetically–different UM cell lines, modified cell lines, or the effect of drugs on tumor growth. The CAM assay is also appropriate for this. One can follow the growth of fluorescent cells in vivo through intravital videomicroscopy, which has been used to analyze the B16F1 melanoma development in chick embryos (Chambers et al. 1992) .
9.3 Other Ocular Tumor Models
9.3.1 Conjunctival Melanoma
Conjunctival melanoma is even rarer than its intraocular “cousin,” and is often associated with many clinical problems, for example, tumors are often multifocal, they often recur locally, they may lack pigmentation, and there are various premalignant noninvasive stages making it difficult to detect and diagnose this disease (Missotten et al. 2005; Shields et al. 2011; Kenawy et al. 2013) . The mutational profile of conjunctival melanoma differs from that of UM and is characterized by BRAF and NRAS mutations in up to 50 and 20 % of cases, respectively (Gear et al. 2004; Spendlove et al. 2004; Lake et al. 2011; Griewank et al. 2013; Weber et al. 2013). Conjunctival melanoma spreads typically via the lymphatics, and at present there are few effective drugs for treatment of these metastases. It is therefore of great importance to develop better therapies, and in order to test drugs for this malignancy, in vitro and in vivo models are required .
While cell culture is often used for testing medications, the availability of an animal model for conjunctival melanoma would be very useful. De Waard recently developed a conjunctival melanoma metastases model (De Waard et al. 2015a, b) . She used three different conjunctival cell lines, of which two have a BRAF (T1799A) mutation (cell lines CRMM-1 and CM2005.1), and one cell line, CRMM-2, an NRAS (A182T) mutation (Nareyeck et al. 2005; Keijser et al. 2007; Gear et al. 2004; Griewank et al. 2013; De Waard et al. 2015a, b) . In vitro grown cells were injected subconjunctivally into genetically–immunodeficient NOD/SCID interleukin (IL)-2 rγnull mice. The three cell lines led to different in vivo characteristics: the primary tumors of CRMM-1 lacked pigment, those of CRMM-2 had some pigment, while the primary tumors of CM2005.1 were heavily pigmented . Although these subconjunctival injections led to tumor growth in all cases, no metastases were seen. Two cell lines grew quite rapidly and when single-cell suspensions were obtained from their subconjunctival xenografts and subsequently implanted subconjunctivally into a second group of mice, metastases ultimately developed .
< div class='tao-gold-member'>
Only gold members can continue reading. Log In or Register a > to continue
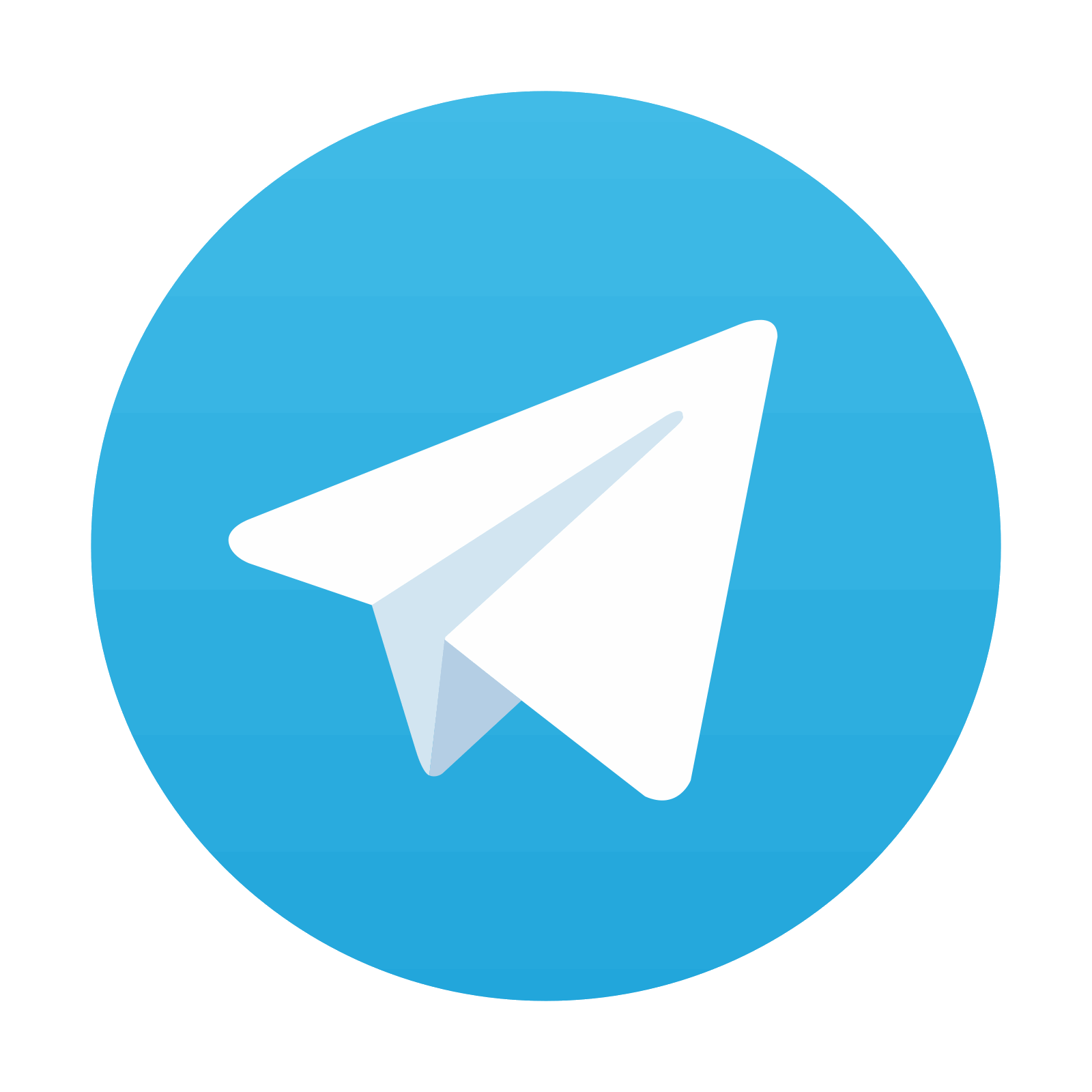
Stay updated, free articles. Join our Telegram channel
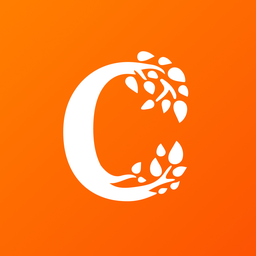
Full access? Get Clinical Tree
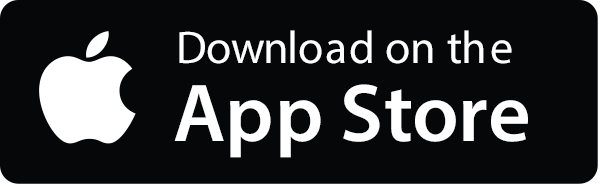
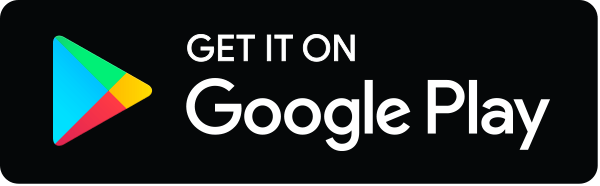