Type of cataract
Animal model/species
Key references cited in review
Congenital/Juvenile
Transgenic
Crystallins
Mouse
11, 12, 13, 17, 18, 20, 52, 53, 55, 173
Rat
137, 138
Zebrafish
45, 77
Connexins
Mouse
47, 122, 134, 161
Structural Proteins
Mouse
2, 16, 99, 125, 131, 132, 133
Rat
158
Diabetic
Induced
Rat
60, 69, 95, 103, 177
Mouse
64, 74, 152
Dog
67
Spontaneous
Rat
89, 97, 106, 127, 130
Dog
174
UV-induced
Rat
35, 72, 80, 94, 139, 140, 151, 156
Mouse
73, 93, 176
Guinea pig
39, 118, 136, 179
Calf
75
Rabbit
40, 43, 179
Steroid induced
Chick embryo
57, 157
Rat
81, 155
Oxygen induced
Rat
42, 76, 116, 178
Guinea pig
4, 41, 50
Rabbit
87, 144
Secondary/ posterior capsular opacification (PCO)
Rat
61, 79, 159
Mouse
85
Chick
153
Bovine
128
Rabbit
58, 59, 110
Dog
19, 24, 114
2.2.1 Crystallins
The crystallin family of proteins makes up the bulk of the protein in the lens, and is the main component for the highly ordered structure that permits the refraction of light. The crystallins can be divided into three classes: alpha, beta, and gamma, named in descending order by average molecular weight when they were first fractionated (Mörner 1894) , each with their own subclass of isoforms. The α-class is coded by two genes CRYAA and CRYAB, and is notable for its chaperone function that protects against protein accumulation associated with temperature change. Several mouse models exist involving mutation of the mouse genes Cryaa (αA) and Cryab (αB). Knockout mouse models were first used to determine the necessity of αA- and αB-crystallin in lens (and general ocular) development; αA-crystallin knockout mice exhibit microphthalmia and nuclear cataracts (Brady et al. 1997), and αA- and αB-crystallin double knockout mice have abnormal fiber cells and no lens sutures (Boyle et al. 2003) . Additionally, αA-crystallin knockout mice have insoluble inclusion bodies inside the lens fiber cells containing αB-crystallin, suggesting that αA has a role in maintaining solubility of αB (Brady et al. 1997). αB-crystallin knockout mice, however, do not develop cataracts and have no significant ocular differences compared with wild type (Brady et al. 2001) . Overall, the mouse knockout models of α-crystallin show that αA is integral in general lens development, as well as in maintaining the regular soluble structure of αB that permits lens transparency.
Mutations in the CRYAA gene were first detected at codon 116, and have been recorded in several different independent populations (Litt et al. 1998; Vanita et al. 2006; Beby et al. 2007; Hansen et al. 2007; Gu et al. 2008; Richter et al. 2008) . Other sites of mutation have been detected in CRYAA in humans, all of which are isolated cataractous phenotypes (Pras et al. 2000; Mackay et al. 2003; Santhiya et al. 2006; Graw et al. 2006) or cataracts accompanied by microcornea (Hansen et al. 2007; Khan et al. 2007; Devi et al. 2008) . Mouse models for four independent point mutations in the Cryaa gene have been described with one mutation in particular, a transition from arginine to cysteine at codon 54, observed in both mouse and humans (Xia et al. 2006b; Chang et al. 1996; Graw et al. 2001) . Interestingly, while the mouse R54C mutation is a dominant mutation, human R54C mutations are inherited as recessive traits, suggesting different functional activities of αA-crystallins in the different species. The αA- and αB-crystallin proteins of the zebra fish have also been investigated, concluding that while the zebra fish αB-crystallin protein is truncated, with lower expression and activity than the human orthologue, the zebra fish and human αA-crystallin proteins are fundamentally similar in structure and activity (Posner 2003) . The zebra fish cloche mutant, which has been used as a model of hematopoiesis and vascular development, also exhibits deficiencies in αA-crystallin that coincided with cataract formation (Goishi et al. 2006) . Decreased levels of αA-crystallin lead to insoluble γ-crystallin that prevent proper fiber cell differentiation, and result in opaque plaques in the lens. Recovery of γ-crystallin solubility upon overexpression of αA-crystallin supports the direct effect of αA chaperone activity of other crystallin proteins, and the necessity of αA-crystallin in lens fiber stability seen in mammalian lenses (Horwitz 2003) .
Unlike α-crystallins, β- and γ-crystallins do not have chaperone function and instead confer more structural roles to the lens . The β- and γ-crystallins each contain two domains that fold into sheets to form a Greek key motif. Repeats of this motif allow dense packing of lens protein that minimizes light scattering. Human β-crystallin has two forms, more acidic and more basic (A and B, respectively), which are coded by six genes CRYBA1,2,4 and CRYBB1,2,3 that are suggested to be the result of gene duplication (Brakenhoff et al. 1992) . The first β-crystallin mutation associated with a disease phenotype was the Philly mouse cataract, in which a long-known inherited cataract in mice (Kador et al. 1980; Zigler 1990) was linked to an altered β-crystallin protein (Nakamura et al. 1988) and finally to a mutation in the Crybb2 gene (Chambers and Russell 1991) . Normal βB-crystallin interacts with other β-crystallin protein in the lens, often in dimers, and the cataract formation caused by the Crybb2 mutation results from the aggregation of mutant βB- with wild-type β-crystallins into insoluble, cataract-forming plaques.
Mutations in the genes encoding βA1/A3-crystallin proteins in mice and humans have also been recorded. Cryba1 and its orthologues encode both the A1 and A3 protein variants of β-crystallin in mammals (Graw 1997) , and wide-scale N-ethyl-N-nitrosourea (ENU)-mutagenesis in mice (Ehling et al. 1985) generated progressive cataracts from mutations in the Cryba1 gene that were with a dominant inheritance pattern (Graw et al. 1999) . In this study, the progression of the nuclear opacity followed the increasing expression of βA1/3-crystallin from E14.5 onward, indicating that the loss of lens transparency was directly proportional to the expression of the mutated protein. A colony of Sprague–Dawley rats with a nuclear cataractous phenotype has also been generated based on an inherited spontaneous mutation in the rat βA1/A3 gene dubbed Nuc1 (Sinha et al. 2005; Sinha et al. 2008) . Although the genetic modifications of knockout mice are not available for replication in the laboratory rat, the rat has an eye that is larger and more complex than the mouse, permitting large-scale study of lenses more representative of human patients. The Nuc1 mutation is a ten base pair insertion into the Nuc1 βA1/A3 gene that causes a frameshift, adding ten new amino acids, interfering with the protein–protein interaction domain (Sinha et al.). Heterozygous Nuc1 mutants have congenital nuclear cataracts , whereas rats with homozygous Nuc1 mutations have prenatal lens rupture and abnormal vascular growth in the eye, including the retention of fetal vasculature and abnormal vascular patterning in the retina (Sinha et al. 2005; Zhang et al. 2005; Gehlbach et al. 2006) . Functional Nuc1 is required for ocular development and necessary to maintain lens homeostasis (Parthasarathy et al. 2011) .
Human γ-crystallin is encoded by six genes; CRYGA to CRYGF, encoding γA–γF-crystallin proteins. The γ-crystallins contain four Greek key motifs that fold to form higher order β-sheets, essential to maintain the transparent lens structure . Mouse models have been used to study the function of γ-crystallin through mutations. The first mutation in a γ-crystallin gene recorded was in the mouse Cryge gene called eye lens obsolescence (Elo) (Cartier et al. 1992) . The Elo mutant is a dominant mutation that alters the reading frame of the fourth Greek key motif, thereby preventing the formation of the highly ordered crystallin structure. The mutant lens fails to develop properly before birth and causes postnatal microphthalmia. Since the Elo mutant, more than 20 independent mouse mutations in Cryg genes have been classified, spanning across all of the six Cryg genes; all are dominant mutations with isolated cataracts as the only resulting phenotype (Graw 2009) . Cataract formation in γ-crystallin mouse mutants involves the formation of amyloid fibrils in the nuclei of lens fiber cells, which disrupt normal transcriptional regulation, first shown in the γBnop-mutant (Sandilands et al. 2002) . These changes have also been observed in wild-type crystallins in lenses subjected to denaturing conditions (Meehan et al. 2004) . It is hypothesized that the main role of α-crystallin chaperone protein is to ensure that the γ-crystallins remain stable, thereby preventing amyloid fibril formation (Hatters et al. 2001) . Further mouse models of crystallin changes indicate accumulations of protein modifications that promote insolubility of the crystallins causing the cataract-forming aggregates develop over time in wild-type mice, similar to the phenotypes of the early-onset mutant models (Ueda et al. 2002) . Modifications to the proteins over time, as well as changes to the lens environment over time, are the factors that contribute to the development of senile cataracts (Wu et al. 2014) . Finally, the zebra fish animal model has also been used for determining the molecular mechanism by which γ-crystallin mutations cause cataracts. An inherited γ-crystallin mutation was found in a three generation Chinese family, Gly129Cys, and the mutation was generated in the zebra fish to observe changes in lens development (Li et al. 2012) .
2.2.2 Connexins
Connexins are gap junction proteins that facilitate exchange of small molecules between cells, particularly important in maintaining homeostasis in avascular tissues such as the lens in which lens transparency relies on a balanced osmotic and metabolic environment (Goodenough 1992) . Three connexin genes expressed in the mammalian lens have been identified, Cx43, 46, and 50, and are encoded by the genes Gja1, Gja3, and Gja8, respectively. Mutations found in the genes Gjp3 (encoding Cx46 or α3) and Gjp8 (encoding Cx50 or α8) have been linked to cataracts with dominant and recessive inheritance. Homozygous Cx46-null mice developed nuclear cataracts that formed due to aggregation of lens protein, facilitated by the degradation of crystallins (Gong et al. 1997) . This mouse model showed normal early lens development, but with small opacities forming 2–3 weeks after birth that continued into full nuclear cataracts by 2 months, with 100 % penetrance of cataracts reported, indicating Cx46 is required for proper lens function (Gong et al. 1997). Interestingly, the severity of the cataracts on the Cx46-null model varied depending on the breeding background of the mouse, with milder cataracts observed in C57B16 mice when compared with mice bred from the 129 strain (Gong et al. 1999) . The insoluble aggregates formed in the nuclear cataracts show disulfide cross-linking between the proteins, resembling human senile cataracts (Garner et al. 1981) .
Mutations in the human Cx50 gene were first linked to cataracts by identifying a missense mutation in a family with inherited cataracts (Shiels et al. 1998) . Since then, more than 15 mutations have been observed in humans at various codons linked to cataracts. In Cx50 homozygous knockout mice, microphthalmia is observed after 2 weeks (White et al. 1998; Rong et al. 2002) . Additionally, small opacities in the lenses were observed at 2 weeks, which continued through 6 months exhibiting clustered nuclear opacities, reflecting the zonular pulverulent phenotype seen in the first observed Cx50 human mutation (Shiels et al. 1998). The Cx50 knockout mouse model suggests not only that Cx50 is required for proper fiber cell protein solubility, but also that Cx50 plays a more general role in lens development, which is known to be correlated with ocular growth (Coulombre and Coulombre 1964) . White et al. replaced Cx50 function by knocking-in Cx46 and found that while the lens development defects of Cx50-null lenses were not corrected by Cx46-induced expression, the cataract phenotype of Cx50-null mice was prevented (White 2002; Martinez-Wittinghan et al. 2003) . The Cx46-replacement mouse model showed that while Cx50 is required for normal lens development, recovery of nonspecific connexin signaling is enough to maintain lens homeostasis lost by knocking out Cx50 and prevent cataract formation. Point mutations have also been detected in the mouse Cx50 gene. Of note, while the heterozygous knockout of Cx50 did not produce a cataract phenotype, individual point mutations of the Cx50 gene in mice lead to dominant cataracts in mice (Mathias et al. 2010) . It is hypothesized that in the formation of the six-membered connexin hemichannels, mutated connexins act as dominant negative inhibitors of wild-type connexins at gap junctions, causing the disruption in homeostasis (Xia et al. 2006a; DeRosa et al. 2007; DeRosa et al. 2009; Berthoud and Beyer 2009; Xia et al. 2012; Rubinos et al. 2014) .
2.2.3 Cytoskeletal and Membrane Proteins
Major intrinsic protein (MIP), also known as aquaporin 0, is a voltage-dependent water channel and the most abundant membrane-associated protein in lens fiber cells (Bloemendal et al. 1972; Gorin et al. 1984) . Models of disruption in the mouse gene Mip determine that the cataractous phenotype of Mip mutant mice is due to disruption in the fiber cell differentiation, and subsequent disruption protein solubility in the crystallin lens (Shiels and Bassnett 1996; Sidjanin et al. 2001; Okamura et al. 2003; Shiels et al. 2001; Al-Ghoul et al. 2003) . The mouse Hfi mutation presents as full total lens opacity in homozygous mice, because of the toxic function of mutated MIP protein. Recently, a rat spontaneous mutation was observed; the KFRS rat model was found to have a spontaneous frame shift mutation in the kfrs4 gene, which inactivates MIP causing recessive cataracts (Watanabe et al. 2012) .
Mutations in structural proteins of lens cells have also been linked to cataracts . Vimentin is a type-III filament that is found in both lens epithelial cell (LEC) and fiber cell and has been shown to interact with α-crystallin (Ramaekers et al. 1980; FitzGerald 2009; Djabali et al. 1997; Nicholl and Quinlan 1994) . Mice have been used successfully to determine the effect of vimentin expression on lens opacity; vimentin knockout mice do not present with cataracts (Colucci-Guyon et al. 1994) , however mice in which a vimentin transgene was used to increase endogenous lens levels of vimentin tenfold developed cataracts (Capetanaki et al. 1989) . In the vimentin overexpressing mice, normal fiber cell differentiation was disrupted and newly formed fiber cells did not elongate or undergo proper denucleation. Similarly, a mouse knockout model of HSF-4, a chaperone factor that represses vimentin overexpression, showed major disruption in lens size and differentiation, resulting in cataracts resembling the vimentin transgenic mice (Mou et al. 2010) . Another structural protein that has been linked to cataract formation is beaded filament structural protein 2. This intermediate filament is present in differentiated lens fiber cells, interacting with α-crystallins and other intermediate filaments, and knockout mice develop opacification (Alizadeh et al. 2002; Sandilands et al. 2003) .
2.3 Models of Age-Related Cataracts
2.3.1 Diabetic Cataract
Diabetes mellitus affects approximately 285 million people worldwide and the International Diabetes Federation reports that this is likely to increase to 439 million by 2030 (Pollreisz and Schmidt-Erfurth 2010) . Premature cataract formation is one of the earliest secondary complications of diabetes and is considered a major cause of visual impairment in diabetic patients. While these cataracts can be successfully treated with surgery, diabetic patients are more susceptible to complications (Stanga et al. 1999) . A complex interaction between genetic factors and environmental factors contributes to the pathogenesis of diabetes in humans; however, this has been difficult to verify (Shiels and Hejtmancik 2007) . The use of experimental animal models has therefore been essential in further resolving these issues and in clarifying the pathogenetic mechanism(s) of diabetic cataract. Experimental diabetes mellitus has been induced using a number of different methods and in multiple animal models (Table 2.1). Most common methods for inducing diabetes involve pharmacological agents such as alloxan or streptozotocin (STZ), which selectively damage the insulin-producing cells in the pancreas resulting in hyperglycemia . Hyperglycemia is the common contributing factor to cataract in both type-1 and type-2 diabetes (Pollreisz and Schmidt-Erfurth 2010) . Using this method, rodents, and in particular rats, have been the most commonly employed experimental model for investigating diabetic cataract. Although larger than mice, rats are still easy to handle and the cost of maintenance is lower than larger mammals. Moreover, diabetes can be induced quickly and effectively in the rat with a single dose of STZ or by galactose feeding resulting in features reminiscent of type-1 diabetes observed in humans. In the STZ model, a substantial (approximately 30 nmol/g lens) accumulation of sorbitol and galactitol occurs in the lens , resulting in the development of “fast” sugar lens opacities (Obrosova et al. 2010) . The high level of sorbitol is thought to initiate osmotic stress in the lens that in turn results in infusion of fluid leading to lens fiber cell swelling and liquefaction. While the specific mechanism(s) by which hyperglycemia causes human cataracts has not been resolved, use of the diabetic rat model has helped to understand some potential, including activation of aldose reductase (AR), an enzyme that catalyzes the reduction of glucose to sorbitol through the polyol pathway, oxidative stress, and the generation of reactive oxygen species (ROS) and nonenzymatic glycation/glycoxidation. More recently, studies using excised rat lenses have also shown that when cultured with high glucose media lenses respond to the osmotic stress by altering the expression of key growth factors such as b-fibroblast growth factor (FGF) and transforming growth factor (TGF) involved in cell signaling, and these may contribute to the cataract formation (Zhang et al. 2012b) .
In addition to the STZ and galactosemic rats, there also exist a number of rat strains that exhibit spontaneous onset diabetes and associated cataracts (Table 2.1). These include the Goto-Kakizaki (Ostenson and Efendic 2007) , Zucker Diabetic Fatty (Schmidt et al. 2003) , Otsuka Long-Evans Tokushima Fatty (OLETF) (Matsuura et al. 2005) , Wistar Bonn/Kobori (WBN/Kob) rats (Mori et al. 1992) and the spontaneously diabetic tori rat (SDT) (Sasase et al. 2013) . In the WBN/Kob rat, plasma glucose levels are elevated at 10 months of age in males only and the cataracts are observed by approximately 12 months. The OLETF rat is characterized by late-onset glycemia, mild obesity, and male inheritance. The AR and sorbitol levels in the lens of this rat strain spike at 40 weeks of age with noticeable cataractous changes at 60 weeks. The SDT is an inbred strain of Sprague–Dawley rat that has gained recent attention since it is the only spontaneous strain that exhibits severe diabetic retinopathy (Sasase et al. 2013). The model also exhibits diabetic cataracts: In the male SDT rats, cataracts are fully penetrant by 40 weeks of age. Opacities are first evident at the posterior pole of the lens and then nuclear sclerosis progresses and the cortex becomes highly opacified. The cataractous lens exhibits features such as swollen lens fibers, liquefaction, vacuolation, abnormal configuration, and the formation of Morgagnian droplets and eventually capsular rupture. Importantly, these complications can be prevented in the SDT rat by normalizing blood glucose with insulin treatment or pancreas transplantation. In addition, the histopathological changes of the lens were preceded by an increase in lens sorbitol content, further suggesting that the cataracts in the SDT rats are a result of sustained hyperglycemia (Sasase et al. 2013) .
Mice are used in many in vivo studies since they are small in size resulting in easier handling and less cost for housing. In addition, the availability of numerous transgenic and knockout strains enables investigators to study the role of a particular gene in disease. However, despite severe hyperglycemia (induced or spontaneous), diabetic mice do not develop cataracts and the levels of AR in the lens remain low (Varma and Kinoshita 1974) . In addition, STZ administration in mice with a single dose, as carried out in the rat, causes lethality typically by 1 month of age, too short of a survival time for the appearance of cataracts. Thus, the mouse has not been a readily used model for diabetic cataract and transgenic models that are used to study other diabetic complications cannot be used to study diabetic cataract. Some attempts have been made, however, with limited success, to create a mouse model of diabetic cataract, by using an STZ multidose method (Hegde et al. 2003) . Additionally, when AR is introduced into the lenses of transgenic mice, sugar cataracts do form under both diabetic and galactosemic conditions, rendering this a potential model (Lee et al. 1995) .
Animal models have also been employed to investigate a number of anti-cataract agents, including pharmaceuticals that could target the various mechanisms underlying diabetic cataract formation, some of which are readily available over the counter medicines or health food supplements. Of these, aldose reductase inhibitors (ARIs) have been explored in great depth, mainly using diabetic models in rat (Kador et al. 2001; Moghaddam et al. 2005; Halder et al. 2003) . A number of plant-derived, natural products known to inhibit AR activity have been explored as well as intrinsic ARI-containing extracts obtained from human kidney and bovine lenses (Kador et al. 2001) . These studies provide evidence that ARIs may prevent and delay diabetic cataract formation.
Experimental induction of diabetes has also been carried out in larger mammals such as the rabbit and the dog (Table 2.1). In the dog, cataract, lens rupture, and lens-induced uveitis are well-known consequences of spontaneous and induced diabetes (Zeiss 2013) . Importantly, the topical ARI drug, Kinostat has shown promise in its ability to reverse early sugar cataract development in dogs (Kador et al. 2006) .
2.3.2 UV-Induced Cataracts
Epidemiological research suggests a strong association between exposure to ultraviolet radiation-B (UVB) and cortical cataract formation in humans (Zigman et al. 1979; Taylor 1980; Delcourt et al. 2000; McCarty and Taylor 2002) . The main UVR source is the sun and because of the fact that the ozone is being depleted, chances of exposure are greater today. While the cornea is the major absorber of UV radiation reaching the eye, UVA and the longest wavelengths of UVB radiation are absorbed by the lens and have been shown to damage lens proteins both in vivo and in vitro (Moreau and King 2012) . In addition, an increase in oxidizing species may occur as a result of UV irradiation . The experimental animal models used to study the effect of UVR exposure on the lens have been quite varied since the lenses of many species are susceptible to UVR-induced cataractogenesis (Table 2.1). Most of the studies involve smaller species such as rats, mice, guinea pigs, and fish. However, rabbits and pigs have also been employed. The majority of these experiments involve anesthetizing the animals, dilating the pupils with mydriatic eye drops, and exposing them to either UVA or UVB of varying dosages, with the most damaging wavelengths located around 300 nm (Pitts 1978; Merriam et al. 2000) . In other cases, however, animals are euthanized, lenses removed, and irradiated in vitro .
The mouse has been used in many UV-induced cataract models in order to elucidate the genetic and biochemical factors at play. For example, transgenic and knockout mice have been used to study the specific role of proteins in protecting the lenses against UV-induced cataracts (Lassen et al. 2007) (Meyer et al. 2009) . As an example, UVB-induced cataract formation was found to be accelerated in mice with single and double knockout of the genes encoding the ALDH1A1 and ALDH3A1, establishing the importance of these two enzymes in the mechanism of cataract formation (Lassen et al. 2007). Furthermore, in these KO mice decreased proteasomal activity, and increased oxidative damage of proteins, was observed in the double knockout mice as compared with wild-type animals. These types of studies can help to further understand how proteins in the lens and cornea can provide added safeguards to prevent UV-induced cataract formation and maintain lens clarity. Recent work using the mouse model has also revealed that older animals are more susceptible to UV-induced cataract formation than young mice (Zhang et al. 2012a) . This may be related to the elevated expression of the thiol damage repair enzymes thioltransferase and thioredoxin found only in young lenses (Zhang et al. 2012a) .
The albino Sprague–Dawley rat has also been a common model over the years with an extensive database on UVB-induced cataract (Galichanin et al. 2010; Soderberg 1990; Soderberg et al. 2002; Wang et al. 2010) . Much of these data, along with other species, have revealed important mechanisms underlying UVR-induced cataract, particularly for UVB and the development of cortical cataract. For example, UVB can cause direct DNA damage and generate ROS, singlet oxygen , superoxide, hydrogen peroxide, and hydroxyl radicals leading to oxidative stress and apoptosis of LECs (Michael et al. 1998; Wang et al. 2010) . UVA and UVB have also been shown to directly damage lens proteins both in vivo and in vitro (Giblin et al. 2002; Simpanya et al. 2008) . Most recent work using the UVB-induced rat model has focused on determining potential anti-cataract/protective agents. For example, caffeine has been shown to have in vitro and in vivo protective effects against UVB irradiation in rats (Kronschlager et al. 2013; Varma et al. 2008) . The lens contains a high concentration of reduced glutathione (GSH), which acts to eliminate oxidants and protect against exogenous and endogenous ROS. Caffeine is thought to protect against UVR-induced cataract by scavenging ROS (Giblin 2000; Lou 2003; Spector et al. 1985; Varma et al. 2008) .
Like humans, lenses of diurnal species, such as duck, frog, guinea pig, rabbit, and squirrel, contain high levels of UVA chromophores, in contrast to lenses of nocturnal species, such as rat and cat (Wood and Truscott 1994; Zigler and Rao 1991) . Thus, these species have been used for studying UVA-induced early nuclear cataract (Giblin et al. 2002) . In particular, studies on guinea pig provide in vivo evidence that link UVA-induced protein aggregation with an increased level of nuclear light scattering . The guinea pig lens is thought to mimic the aging human lens because it possesses a high level of a protein-bound toxic chromophore—not protein-bound kynurenine as in the aging human lens (Parker et al. 2004) , but protein-bound NADPH in the form of zeta crystallin, which makes up 10 % of total lens protein in the guinea pig (Rao and Zigler 1990; Zigler and Rao 1991) . Presumably, this along with trace amounts of molecular oxygen leads to UVA-induced generation of ROS. A similar process of UVA-induced protein aggregation may take place in the older human lens nucleus, possibly accelerating the formation of human nuclear cataract. Finally, UVA exposure has also been shown to induce cataracts in calves (Lee et al. 1999) and in vitro cataract development in pig lenses (Oriowo et al. 2001) .
The rabbit lens also contains high levels of the UVA-absorbing pyridine nucleotide NADH, both free and bound to l-crystallin (Giblin and Reddy 1980; Zigler and Rao 1991) . Many of the rabbit studies investigate the preventive effect of contact lenses containing UV-blocking materials . These findings show that contact lenses are beneficial in protecting ocular tissues of the rabbit against harmful effects of UV light, including photokeratitis and anterior subcapsular cataract (Giblin et al. 2012) .
2.3.3 Steroid-Induced Cataracts
The use of steroid therapy in recent years has contributed to an increase in the incidence of cataracts (Gupta and Wagner 2009; Watanabe et al. 2000) . Administration of synthetic glucocorticoids (GCs), or corticosteroids, as anti-inflammatory or immunosuppressive agents have been used in the treatment of many diseases including rheumatoid arthritis and asthma as well as in organ transplants and chemotherapy. The cataracts formed by steroid use typically occur in the posterior region of the lens, beneath the lens capsule and are thus referred to as posterior capsular cataracts (PSC) (Eshaghian and Streeten 1980) . PSCs exhibit swollen fiber-like cells in the posterior pole of the lens, which have retained nuclei. The association between steroid use and the formation of cataracts in humans was made in the 1960s following the examination of 72 patients being treated for rheumatoid arthritis (Black et al. 1960) . In all, 42 % of the patients developed cataracts whereas none of the patients in the control group did. Despite knowing GCs induce PSC, either through topical or systemic treatment, it remains unknown whether the effect is due to direct action on lens cells by binding to glucocorticoid receptors (GRs) or by indirect mechanisms .
A small number of animal models have been developed to study the underlying causes of steroid-induced cataracts (Table 2.1). The chick embryo has been used to study the response of the lens to GC. GRs have not been identified in the chick, and thus, GCs are thought to act on lens cells indirectly. It has been proposed that they do so by binding to GRs in the liver resulting in an increase in blood lipid peroxides that travel to the aqueous humor and deplete the lens of glutathione (Gupta and Wagner 2009; Watanabe et al. 2000). This in turn is thought to result in an increase in oxidative stress and formation of cataracts (Gupta and Wagner 2009; Watanabe et al. 2000) . These findings are somewhat paradoxical, however, since treatment of chick lenses alone with GC can also develop cataracts, suggesting that additional mechanisms are at play in GC-induced cataract formation .
More recently, the mammalian lens has been shown to express GR, suggesting that direct activation by GC may also cause PSC formation. For example, the development of PSC in rabbits and rats treated with GC has been reported. Rats have been used more extensively as a mammalian model for exposure to high-dose steroids and this resulted in altered expression of the cell adhesion molecules, E- and N-cadherin, suggesting this may contribute to the disruption in fiber cell differentiation observed in PSC (Lyu et al. 2003) . To further explore the mechanism of response of lens cells to GC rat ex vivo lens explants have also been used. This study demonstrated that treatment of explants with the GC, dexamethasone, along with the growth factor, fibroblast growth factor 2 (typically found in the aqueous and vitreous humors), resulted in an increase in cell proliferation and coverage on the capsule, occurrences observed in PSC formation (Wang et al. 2013) . Future research has been proposed using the rat model to explore potential signaling pathways induced by GC including ERK1/2, known to be involved in fiber cell differentiation (Wang et al. 2013) . Some caution has been made, however, that some differences in steroid-induced signaling may occur in rats as compared with the human lens because of the fact that other receptors are present in the human lens such as the GR receptor (Gupta and Wagner 2009) . Thus, it was suggested that findings in the rat should be verified with using human lenses (Gupta and Wagner 2009) .
2.3.4 Oxygen and Nuclear Cataracts
Typically, the lens resides in a hypoxic environment and increased exposure to oxygen is thought to be a significant cause of age-related nuclear cataracts (Beebe et al. 2010a) . For example, it has been shown that individuals who received hyperbaric oxygen (HBO) therapy for more than 1 year developed nuclear cataracts (Beebe et al. 2010a; Palmquist et al. 1984) . Additional evidence to support this comes from patients undergoing vitrectomy, which involves the destruction and removal of vitreous humor, as is typically done during retinal surgery. Following vitreous removal the lens is exposed to high levels of oxygen and these patients exhibit a high incidence (60–95 %) of nuclear cataract formation (Cherfan et al. 1991; de Bustros et al. 1988; Thompson et al. 1995) . The intact biochemical nature and gel structure of the vitreous are thought to protect the lens from oxygen and nuclear cataract formation. With age the vitreous undergoes collapse, referred to as vitreous syneresis, and loses its ability to maintain a low oxygen tension around the lens (Beebe et al. 2010a) .
Animal models have been used to both measure oxygen levels in the vitreous and determine the mechanism of how high oxygen levels cause cataract formation (Table 2.1). Initially, studies using animal models confirmed the effect of high oxygen exposure on nuclear opacification. The main model for this has been the guinea pig, which when exposed to multiple treatments of HBO over extended periods of time exhibited lenses with increased nuclear scatter, along with protein insolubilization and increased levels of oxidized glutathione in the lens nucleus (Giblin et al. 1995; Bantseev et al. 2004; Gosselin et al. 2007) . Studies using both cats and rats have shown the importance of the vitreous in protecting the lens from increased oxygen levels. For example, when the cat vitreous is disrupted enzymatically increased oxygen levels occur. Rats exposed to HBO along with a loss of the vitreous resulted in decreased levels of protective enzymes in the lens and an increased level of glutathione protein mixed disulfides, unlike those animals in which the vitreous was left intact (Quiram et al. 2007; Giblin et al. 2009; Li et al. 2013) . Further research using rats has shown that when hyperoxic conditions induce nuclear cataracts this is associated with mitochondrial DNA damage (Zhang et al. 2010) . Some investigators have developed synthetic gels to replace the vitreous after vitrectomy and these studies have been primarily carried out in primates and rabbits (Maruoka et al. 2006; Swindle-Reilly et al. 2009) . These studies were designed to help in re-attaching the retina after detachment and further studies are needed to determine if they can restore low oxygen tension at the surface of the lens .
2.4 Secondary Cataract
Secondary cataract, also known as PCO, is one of the most common complications following cataract surgery. In PCO, LECs, which remain within the capsule after cataract surgery, are triggered to proliferate and transdifferentiate (West-Mays and Sheardown 2010; Wormstone et al. 2009) . Cells derived from the anterior lens epithelium, referred to as “A cells,” are those thought to transdifferentiate into spindle-shaped myofibroblasts through a process known as epithelial-to-mesenchymal transformation (EMT) (West-Mays and Sheardown 2010; Wormstone et al. 2009). These cells migrate to the posterior lens capsule where they deposit matrix and cause wrinkling of the capsule, resulting in disruption of vision. PCO was initially diagnosed following the beginning of extracapsular cataract extraction (ECCE) and remained fairly common during these early days (late 1970s and early 1980s) with incidence in up to 50 % of patients (Bullimore and Bailey 1993; Kappelhof and Vrensen 1992) . However, advances in intraocular lens (IOL) design and surgical technique over the past 20 years have resulted in a dramatic reduction in reported PCO rates, to occurrence in 14–18 % of patients (Schaumberg et al. 1998; Wormstone 2002; West-Mays and Sheardown 2010; Wormstone et al. 2009) . Yet, PCO remains a major medical problem with profound consequences for the patient’s well-being and is a significant financial burden due to the costs of follow-up treatment .
< div class='tao-gold-member'>
Only gold members can continue reading. Log In or Register a > to continue
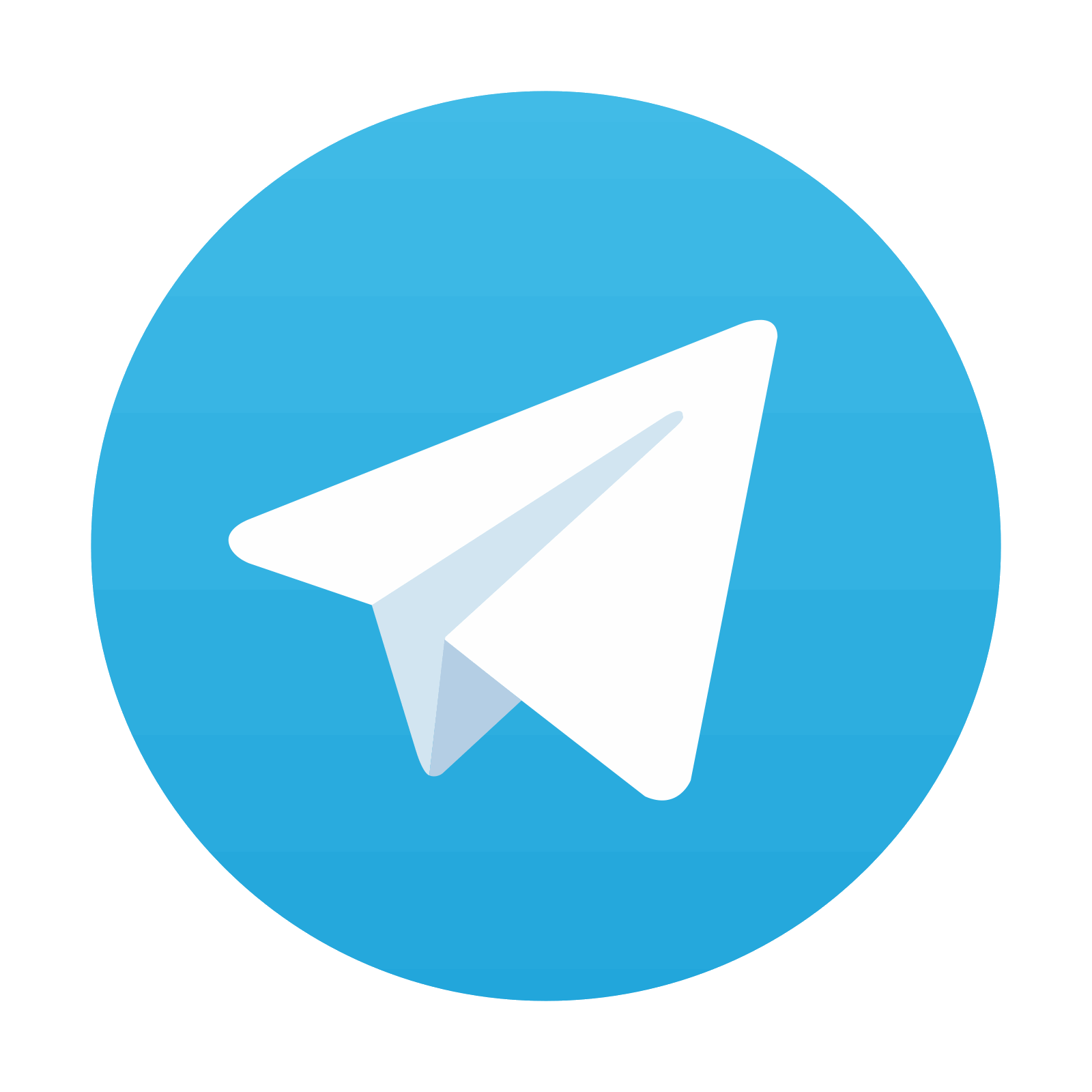
Stay updated, free articles. Join our Telegram channel
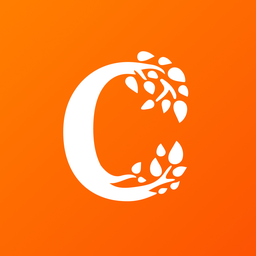
Full access? Get Clinical Tree
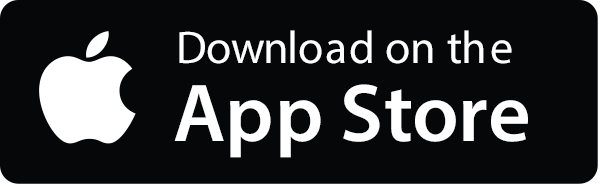
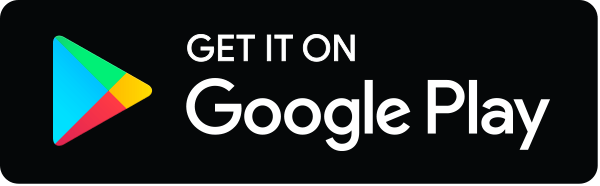