© Springer International Publishing Switzerland 2016
Chi-Chao Chan (ed.)Animal Models of Ophthalmic DiseasesEssentials in Ophthalmology10.1007/978-3-319-19434-9_44. Animal Models of Age-Related Macular Degeneration: Subretinal Inflammation
(1)
Inserm, U 968, UPMC University Paris 06, 75012 Paris, France
(2)
UMR_S 968, Institut de la Vision, UPMC University Paris 06, 75012 Paris, France
(3)
INSERM-DHOS CIC 503, Centre Hospitalier National d’Ophtalmologie des Quinze-Vingts, 75012 Paris, France
4.1 Introduction
Ideally, an animal model of a given human disease should be triggered by the same risk or causative factors observed in humans and lead to a similar pathology. This is, to a large degree, the case in monogenic disease, when transgenic animals bearing the human genetic mutation (and not the knockout of the whole corresponding gene) develop a similar pathology observed in humans. We could call these models “first-degree” models as they rather precisely reproduce the origin and ensuing pathomechanism of a given disease. These are ideal models to decipher how the causative mutation alters molecular and cellular homeostasis and leads to tissue alterations and disease. Most diseases, however, are complex and caused due to several factors that are often not all known. In these cases the chronic interplay of several risk/causative factors leads to secondary and tertiary events such as the death of certain cell population, accumulation of waste products and debris, inflammation, and/or the uncontrolled proliferation of certain cell types (endothelial cells, fibrous tissue). Animal models can be designed to mimic certain downstream stages of the disease and decipher subsequent mechanisms. “Downstream” models usually reproduce only one or a limited number of phenotypes/symptoms of the complex disease which are often not specific to the complex disease. They are by definition more problematic, as they induce a phenotypically similar phenotype in animals by other means than the risk/causative factors that are responsible for the human disease. They can be useful to understand downstream mechanisms that induce the similar phenotype but have to be interpreted with caution as distinct mechanisms might lead to the same phenotype.
Age-related macular degeneration (AMD) is a common, complex disease that results from interplay of genetic and environmental risk factors. Polymorphisms in the complement factor H (CFH, an inhibitor of the alternative complement cascade), the promotor of the high-temperature requirement A serine peptidase 1 (HTRA1, a serine protease involved in inflammation) , and the apolipoprotein E isoforms (ApoE, a lipoprotein involved in reverse cholesterol transport and immune regulation) likely account for a large part of the genetic risk to AMD (Swaroop et al. 2007). Age and smoking are the main environmental risk factors for AMD, but other factors such as poor diet and excessive light exposure likely play a role (Chakravarthy et al. 2010). Given the multifactorial nature of the disease and that none of the risk factors on its own is sufficient to trigger the disease in humans, it is to be expected that animal models mimicking a single factor, or a combination of only two risk factors, is not sufficient to produce all aspects of AMD. However, these models might be able to reproduce different features of AMD and provide an entry point into the understanding of the pathomechanism and the identification of potential drug targets.
Subretinal inflammation is observed in early/intermidate, and late AMD (see below). It is clearly not specific to AMD, but this is also true for drusen, photoreceptor/retinal pigment epithelium (RPE) cell death, and choroidal neovascularization (CNV) as they are all individually observed in other diseases and are the manifestations of ill-defined dysfunctions. We will also see in this chapter that some “first-degree” animal models of AMD-risk factors suggest that impaired subretinal immune suppression and inflammation is one of the initiating events in AMD. Primary or secondary, non-resolving, chronic inflammation often becomes pathogenic and contributes to disease progression, as seen in many chronic inflammatory diseases (Nathan and Ding 2010). To understand the initiation of chronic subretinal inflammation and its possible pathogenic role in AMD, we are greatly dependent on animal models. In this chapter we first summarize the evidence and nature of subretinal inflammation in AMD. We next review whether and how known AMD risk factors influence eventual subretinal inflammation in “first-degree” AMD animal models. We next outline “primary” inflammation models, where the subretinal inflammation is due to a defect in tonic anti-inflammatory signals, deficient leukocyte elimination, or an autoimmune reaction. Last but not least, we give an overview on “secondary” inflammation models, where the subretinal inflammation occurs after a primary injury or degeneration. We concentrate on mouse models, as the vast majority of research in the field is currently carried out on rodent models .
4.2 Inflammation and Age-Related Macular Degeneration
Inflammation is a frequent occurrence in the context of tissue injury (sterile inflammation) and microbial infection. Mononuclear phagocytes (MPs) play a central role in the inflammatory reaction. MPs comprise a family of cells that include monocytes (Mos), inflammatory and resident macrophages (iMφ, rMφ), microglial cells (MC, the rMφ of the central nervous system) and dendritic cells (DC; Gautier et al. 2012). MPs survey the local tissue environment, and are involved in the innate and adaptive immune responses that occur during inflammation. Inflammation is typically characterized by rMφ/MC activation, early neutrophil recruitment followed by an influx of Mos that differentiate into iMφ (Wynn et al. 2013; Nathan and Ding 2010). They secrete a variety of inflammatory mediators which activate antimicrobial defense mechanisms, including oxidative processes and complement activation that contribute to the killing of invading organisms (Wynn et al. 2013). Some iMφs and inflammatory DCs can migrate from the injury site to the draining lymph nodes (LNs), where they initiate an adaptive immune response. At the injury site, neutrophils undergo death within hours and are cleared together with pathogens and tissue debris by macrophages . Phagocytosis triggers the production of mediators which generate macrophages that facilitate tissue repair and/or scar formation and resolution (Fadok et al. 2001; Huynh et al. 2002). Finally, iMφs disappear from the site and the tissue is left with the tissue-specific rMφ (Gautier et al. 2013). Ideally, inflammation quickly and efficiently eliminates pathogens and repairs the tissue injury either by regeneration or by scarring. If the inflammatory response is not quickly controlled, it can become pathogenic and contribute to disease progression , as seen in many chronic inflammatory diseases (Nathan and Ding 2010).
The inflammatory response consists of a compromise of fast effective elimination and neutralization of pathogens, and avoidance of collateral tissue damage that interferes with vital functions. The retina is especially vulnerable to immunopathic damage as it has very limited regenerative capacities. A blood–tissue barrier and a physiological immunosuppressive environment particularly protect it from excessive leukocyte infiltration. Thereby, innate and adaptive immune responses are dampened or suppressed, possibly to minimize collateral damage of the inflammatory response (Streilein 2003).
Physiologically, the posterior segment of the eye contains numerous rMφs in the choroid and ciliary body and some perivascular rMφs in the retina. A network of MCs is located in the inner retinal layers, but a number of neuron- and glial-derived factors continually represses their activation (e.g., CX3CL1, CD200L, SIRP 1α, CD22; Galea et al. 2007). The central subretinal space, located between the RPE and the photoreceptor outer segments (POS), is physiologically devoid of MPs in healthy subjects (Gupta et al. 2003; Combadiere et al. 2007; Sennlaub et al. 2013), and they are only present in the extreme periphery (McMenamin and Loeffler 1990). The immunosuppressive capacities of the healthy RPE likely contribute to this peculiarity: the RPE induces apoptosis of lymphocytes and iMφs efficiently. The immunosuppressivity of the RPE is well illustrated by the fact that RPE allografts to nonimmune privileged sites of non-immuno-compatible hosts can survive prolonged periods of time (Wenkel and Streilein 2000) . Furthermore, the retina has no lymphatic drainage system and only a few DCs (Streilein 2003; Forrester et al. 2010; Kumar et al. 2014), and the subretinal space lacks blood vessels through which effector cells could infiltrate the tissue (Streilein et al. 2002).
Early and intermediate AMD is characterized by sizeable deposits of lipoproteinaceous debris, called large drusen, located in Bruch’s membrane (BM) and partially covered by the RPE (Sarks 1976). The presence of large drusen is an important risk factor for late AMD (Klein et al. 2004). There are two clinical forms of late AMD: wet AMD, which is defined by CNV, and geographic atrophy (GA), which is characterized by an extending lesion of both the RPE and photoreceptors (Sarks 1976) .
The first histological evidence of subretinal leucocytes in exudative AMD dates back to 1916 (Hegner 1916) and has been reproduced in numerous publications since (Penfold et al. 2001). In particular MPs and lymphocytes are invariably observed in excised neovascular membranes from patients with AMD (for review see (Penfold et al. 2001)). In GA, MPs have been observed within the atrophic area (Gupta et al. 2003; Combadiere et al. 2007; Penfold et al. 2001; Levy et al. 2015), on RPE cells adjacent to the lesions (Gupta et al. 2003; Sennlaub et al. 2013; Levy et al. 2015), and within and around drusen (Sennlaub et al. 2013; Levy et al. 2015; Killingsworth et al. 1990; Hageman et al. 2001).
Most of the published studies concerning MP accumulation in AMD do not permit the differentiation of distinct types of MPs, such as Mo-derived iMφs and MCs, as they are morphologically indistinguishable and express or induce similar markers (Gautier et al. 2012; Ransohoff and Cardona 2010). Using CCR2 as a specific marker, of iMos/iMφs (Geissmann et al. 2010), as it not expressed and cannot be induced in MCs (Saederup et al. 2010), we have recently shown that inflammatory CCR2+ Mos/iMφs invariably take part in the subretinal inflammation in GA lesions and large drusen (Sennlaub et al. 2013).
Lymphocytes are rarely observed in the retina of AMD patients, but infiltrate the choroid and neovascular membranes (Penfold et al. 2001). Furthermore, interleukin (IL) 17A, a pro-inflammatory cytokine and major product of CD4+ T helper 17 lymphocytes is increased in eyes with GA (Wei et al. 2012). A recent report also shows an increased number of degranulated mast cells in the choroid of GA patients (Lutty et al. 2013) .
The inflammation in AMD is relatively mild and a neutrophil infiltration has not been described, compared to fast evolving autoimmune retinitis lesions, in which an invasion of cytotoxic T lymphocytes, neutrophils, and macrophages are observed (Kerr et al. 2008). This difference might reflect the slow progression of the degeneration bserved in GA compared to fast tissue destruction that characterizes chorioretinitis.
To date, it is not clear if the observed inflammatory changes are purely a result of degenerative and neovascular changes and do not influence yje disease, if they initiate AMD or participate in AMD progression, or if they are protective for the tissue. The observation that chorioretinal inflammation can be sufficient to cause CNV in young patients with chorioretinits in the absence of prior degenerative changes, and that immunosuppressive agents in wet AMD patients lower the need for anti-vascular endothelial growth factor (VEGF) therapy (Nussenblatt et al. 2010) suggests that the inflammation can be pathogenic at least in exudative AMD (for a review of anti-inflammatory agents in AMD see Wang et al. (Wang et al. 2011)).
Animal models of subretinal inflammation can help to further elucidate whether and how AMD risk factors influence subretinal inflammation, and whether subretinal inflammation participates in CNV and degeneration.
4.3 Subretinal Inflammation in AMD Animal Models
4.3.1 AMD-Associated Risk Factors and Inflammation
4.3.1.1 Genetic Risk Factors
The common polymorphisms in CFH, HTRA1, and the ApoE isoforms account for a large part of the genetic risk to late AMD (Swaroop et al. 2007). They are also associated with early/intermediate AMD (Yu et al. 2012), which might signify that they are not specific to RPE/photoreceptor degeneration or CNV, but implicated in pathomechanisms that are common to early/intermediate and late AMD. Such a common mechanism could be subretinal inflammation but it is not yet clear whether or how the disease-associated alleles influence subretinal inflammation . Interestingly, all three proteins are expressed at high levels in activated MPs (HTRA1 (Hou et al. 2013), ApoE (Levy et al. 2015; Peri and Nusslein-Volhard 2008; Basu et al. 1982; Rosenfeld et al. 1993), and CFH (Griffiths et al. 2009; Calippe et al. 2014)) and might be directly involved in MP function.
A common polymorphism or isoform appears in a population, when a germ line mutation in the ancestral version of an allele generates an advantage in the “fitness” (survival, reproduction, etc.) of its carrier. The frequency of the allele thereby increases over the generations in the population under evolutionary pressure. Infectious diseases exert a constant, strong evolutionary pressure on the genetic makeup of a population. The AMD-associated polymorphisms might have been selected because they lead to a stronger inflammatory response and better defense against infectious disease.
ApoE, for example, plays a role in susceptibility to infection (Roselaar and Daugherty 1998; de Bont et al. 1999; Sinnis et al. 1996) and APOE3 allele carriers are somewhat protected against progression and death from HIV infection (Burt et al. 2008) compared with the APOE4 genotype, believed to be the ancestral isoform (Raichlen and Alexander 2014). On the other hand, APOE3-allele carriers carry an increased risk for AMD compared with APOE4-allele carriers, which further increases in APOE2-allele carriers (McKay et al. 2011). The APOE2 isoform is associated with increased ApoE transcription and protein levels in humans and in humanized transgenic mice that express the APOE2 allele (Yu et al. 2013; Mahley and Rall 2000). In mice, we have recently shown that ApoE overexpression in MPs, observed in Cx3cr1-deficient mice, downregulates RPE FasL expression via IL-6 and leads to prolonged survival and accumulation of subretinal MPs (Levy et al. 2015). Overexpression of ApoE in APOE2 mice might lead to a similar effect and promote subretinal MP accumulation. APOE2 mice might present a “first-degree” AMD model for subretinal inflammation in the future.
Similarly, the AMD-associated 402H CFH, which alters CFH surface binding depending on the nature of the surface (Clark et al. 2006), binds significantly less to certain pathogenic bacteria that have evolved the capacity to bind CFH and escape complement-mediated elimination (Haapasalo et al. 2008). The 402H allele was proposed to have emerged in the European population because it was a survival factor during bacterial pandemics (Clark et al. 2010). To our knowledge, most studies that analyzed CFH location in AMD have shown that CFH is increased in the choroid and in drusen of AMD patients (for a review see Calippe et al. (Calippe et al. 2014)). Interestingly, the transgenic expression of CFH under the APOE promoter, which likely leads to increased CFH expression in the eye where ApoE is strongly transcribed, develops significant age-dependent subretinal inflammation compared with control mice, suggesting that CFH is involved in subretinal MP accumulation (Ufret-Vincenty et al. 2012). However the increased accumulation was independent of the polymorphism and better models, such as CFH H knockin mice, are needed that precisely replicate the human risk allele to determine whether and how the CFH polymorphism directly affects subretinal inflammation.
Last but not least, HTRA1, which promotes inflammation (Grau et al. 2006), is increased in carriers of the AMD-associated single nucleotide polymorphism (SNP; Yang et al. 2006; Chan et al. 2007), and transgenic mice that overexpress HTRA1 have been reported to present a subretinal MP accumulation (Liao et al. 2013).
Taken together, the three main AMD-associated alleles affect proteins involved in inflammation and might have been selected in certain populations due to the evolutionary pressure of infectious diseases. Animal models that more or less accurately mimic the AMD-associated allele or allele effect appear to promote subretinal inflammation. It will be interesting to see whether humanized knockin mice that express the AMD risk alleles develop a similar phenotype.
4.3.1.2 AMD-Associated Environmental Risk Factors and Inflammation
Age and smoking are the main environmental risk factors for AMD (Chakravarthy et al. 2010), but other factors such as the patient’s pigmentation and excessive light exposure might also play a role (Klein et al. 2014). Age has been shown to be sufficient to induce subretinal accumulation of MPs in wild-type (WT) mice (Xu et al. 2008). Similarly, conventional, nontoxic light (250 lux) is enough to induce subretinal MP accumulation in albino mouse strains, but not in pigmented mice (Ng and Streilein 2001). The fact that subretinal MPs do not accumulate when the albino mice are raised in darkness and are eliminated when placed into darkness clearly shows the light dependence of the accumulation in these albino mouse strains. Age and/or light exposure are also essential in the accumulation of subretinal MPs in “primary inflammation” models (see below). To our knowledge there are no studies investigating a subretinal inflammation in experimental chronic cigarette smoke exposure to date, but smoking has been shown to exacerbate a variety of auto-inflammatory and autoimmune diseases (Arnson et al. 2010).
Taken together, several animal models that mimic individual AMD risk factors are sufficient to induce subretinal MP accumulation but not degeneration or CNV, which might suggest that subretinal inflammation is an early feature in the pathogenesis. It remains to be seen whether one model that unites all known genetic and environmental risk factors leads to more severe subretinal inflammation that could trigger degeneration and CNV and constitute an ideal model of AMD.
4.3.2 „Primary inflammation“ AMD Models
Several animal models of subretinal inflammation exist, in which the inflammation is not a secondary event to photoreceptor or RPE dysfunction but due to (i) deficient physiological tonic anti-inflammatory signals, (ii) deficient immunosuppressive environment, or (iii) the induction of an autoimmune response to subretinal epitopes.
4.3.2.1 Suppression of Tonic Anti-Inflammatory Signals
Physiologically, neurons express a number of factors that continually repress MC activation, such as CX3CL1, CD200L, SIRP 1α, and CD22 (Galea et al. 2007). In the retina, CX3CL1 is constitutively expressed as a transmembrane protein in inner retinal neurons (Zieger et al. 2014) and provides a tonic inhibitory signal to CX3CR1-expressing retinal MCs that keeps these cells in a quiescent surveillance mode under physiological conditions (Ransohoff 2009). Deletion of Cx3cr1 leads to an accelerated age-dependent increase of subretinal MPs in pigmented animals in both Cx3cr1−/− knockout (Combadiere et al. 2007) and Cx3cr1GFP/GFP knockin mice (Combadiere et al. 2007; Sennlaub et al. 2013; Levy et al. 2015; Chinnery et al. 2011) compared with WT animals kept under the same light conditions (~ 250 lux). The phenotype is not due to the Crb1rd8 contamination (see below) as the mice were not contaminated in these experiments. Cx3cr1 deletion also leads to a significant accumulation in young albino mice (Combadiere et al. 2007; Chinnery et al. 2011) and a light challenge (that is not itself toxic, as it does not induce subretinal inflammation or degeneration in control mice) induces MP accumulation in pigmented Cx3cr1GFP/GFP mice (Sennlaub et al. 2013; Levy et al. 2015). Raising albino Cx3cr1−/− mice in darkness (Combadiere et al. 2007) or letting pigmented C57BL/6 Cx3cr1GFP/GFP mice age in very dim light conditions prevents the accumulation (Luhmann et al. 2013; Combadiere et al. 2013), which confirms the importance of light in subretinal MP accumulation (Ng and Streilein 2001). Strikingly similar to CX3CL1, CD200 is expressed by neurons of the inner retina and provides a tonic inhibitory signal via the CD200R receptor expressed by MPs (Broderick et al. 2002). Cx3cr1−/− and CD200R−/− mice develop exaggerated subretinal MP accumulation and CNV (Combadiere et al. 2007; Horie et al. 2013). Furthermore, the age-, light-, and laser-dependent accumulation of subretinal Cx3cr1-deficient MPs is associated with a significant loss in photoreceptors (Combadiere et al. 2007; Chinnery et al. 2011). Mechanistically, we showed that the lack of the tonic inhibitory signal of CX3CR1 leads to increased expression of CCL2 and recruitment of neurotoxic blood-derived iMφs under the retina , also observed in GA patients (Sennlaub et al. 2013). Furthermore, Cx3cr1-deficient MPs of all origins studied (peritoneal Mφs, bone marrow derived Mos, and brain MCs) are more resistant to clearance after subretinal adoptive transfer to WT recipients (Levy et al. 2015). We showed that APOE-induced IL-6 release from the MPs represses RPE FasL-mediated immune-suppression, and prolongs subretinal MP survival (Levy et al. 2015). Taken together the increased survival (Levy et al. 2015) and recruitment (Sennlaub et al. 2013) likely explains the accumulation of subretinal MPs observed in these animals.
In summary, Cx3cr1-deficient mice do not develop drusen or RPE atrophy, but provide a model of “primary” subretinal MP accumulation (when observed with age or in a nontoxic light-challenge model) that is associated with photoreceptor degeneration. In this model the subretinal inflammation develops under conditions that do not cause direct damage to photoreceptors or the RPE and the initiating cause lies within the MPs, as CX3CR1 is expressed by MPs but not by photoreceptors or RPE cells. It is particularly useful to decipher the mechanisms of MP accumulation and the effect of subretinal MPs on photoreceptor homeostasis, as the phenotype is not complicated by other reasons for photoreceptor death (such as genetic defects or toxic light conditions that directly induce cell death). The model can also be used to accentuate “secondary” models, such as a laser injury where they develop excessive inflammation and CNV, characteristics they share with CD200R−/− mice. Cx3cr1-deficient mice also display increased CCL2, ApoE, and IL6 expression observed in AMD (Sennlaub et al. 2013; Levy et al. 2015; Jonas et al. 2010; Anderson et al. 2001; Klaver et al. 1998; Klein et al. 2008; Seddon et al. 2005) and might therefore share important pathogenic deregulations with the human disease. Furthermore, Cx3cr1 polymorphisms have been associated with wet AMD in some studies (Combadiere et al. 2007; Anastasopoulos et al. 2012; Tuo et al. 2004) and CX3CL1 expression decreases with age in the central nervous system (Fenn et al. 2013). These results suggest that altered CX3CL1/CX3CR1 signaling might be directly involved in the athogenesis.
4.3.2.2 Defective Immunosuppressive Environment
The healthy RPE has strong immunosuppressive capacities (Wenkel and Streilein 2000) and induces MP apoptosis in vivo, evidenced by the fast clearance of MPs adoptively transferred to the subretinal space (Levy et al. 2015). In GA, MPs not only accumulate within the lesions, where the RPE is missing (Gupta et al. 2003; Combadiere et al. 2007; Penfold et al. 2001; Levy et al. 2015), but also on the RPE cells adjacent to the growing lesion (Gupta et al. 2003; Sennlaub et al. 2013; Levy et al. 2015) and on and around large drusen (Sennlaub et al. 2013; Levy et al. 2015; Killingsworth et al. 1990; Hageman et al. 2001). This accumulation might be of pathomechanistical importance as activated MPs have been shown to be able to induce RPE and photoreceptor cell death and might participate in lesion growth in GA (Sennlaub et al. 2013; Yang et al. 2011). The RPE immuno-suppressive mediators that physiologically participate in the elimination of subretinal MPs and prevent accumulation are ill defined. However, several animal models appear to present subretinal MP accumulation secondary to a defect in the RPE immunosuppressive capacities. The RPE constitutively expresses FASL (CD95L), which in part mediates its immunosuppressive characteristics (Wenkel and Streilein 2000) . Indeed, FASL-defective- ( FasL gdl/gld -mice), and FAS-defective-( Fas lpr/lpr -mice) mice develop significant subretinal MP accumulation after a light challenge not strong enough to cause inflammation or damage in WT mice, similar to Cx3cr1 GFP/GFP mice (Levy et al. 2015). This increase in subretinal MPs is likely the result of deficient elimination, as subretinally injected MPs survived better, when FasL/FAS signaling was defective ( WT MPs injected in FasL gld/gld -mice and Fas lpr/lpr -MPs injected in WT mice compared with WT MPs injected in WT mice) (Levy et al. 2015). Similarly, subretinal MPs have been described to accumulate in uninjured TSP-1−/− mice (Ng et al. 2009) . One might suspect that the Crb1rd8 mutation was present in the mice used for this report, as the typical retinal lesions are depicted in the figures (also see below). However, we recently reported the same phenotype in Crb1rd8-free, light-challenged TSP-1−/− mice (Levy et al. 2013). Similar to FAS/FASL deficiency, the clearance of subretinally injected MPs was impaired when TSP-1 was absent and restored by recombinant TSP-1. Interestingly, TSP-1 expression has been shown to be decreased in AMD (Uno et al. 2006) and its diminished expression might participate to create an inflammation permissive microenvironment.
Taken together, FasL gld/gld -, Fas lpr/lpr -, and TSP-1−/− mice likely represent animal models in which subretinal inflammation develops due to a weakened subretinal immunosuppressive environment. There are likely other factors that play a role and future research will hopefully complete the picture .
4.3.2.3 Autoimmune Reaction
AMD shares several similarities with classical autoimmune diseases such as lymphocyte accumulation and autoantibody formation. In particular, carboxyethylpyrrole (CEP) autoantibodies have been shown to be increased in AMD plasma (Gu et al. 2010) and CEP-modified proteins accumulate in the outer retina and drusen in AMD (Crabb et al. 2002). CEP are protein modifications that form from an oxidation fragment of docosahexaenoic acid (DHA). Intriguingly, an immunization of B57BL6 mice with CEP-modified mouse serum albumin (CEPMSA) induced a CEP-specific immune response, subretinal inflammation , and focal lesions of the RPE such as vesiculation (Hollyfield et al. 2008). Furthermore, a CEP immunization of BALB/c mice was sufficient to induce subretinal MP accumulation, followed by RPE lesions and finally photoreceptor cell loss, the main features of GA (Cruz-Guilloty et al. 2013). Interestingly, the main effector cell in this autoimmune model was shown to be CCR2+ Mo-derived iMφs, rather than a cytotoxic T-cell response (Cruz-Guilloty et al. 2013).
In summary, the above-described animal models are “primary inflammation” models, in the sense that the subretinal inflammation develops between originally healthy photoreceptors and RPE cells and under living conditions that do not directly cause RPE or photoreceptor injury. They are particularly useful to decipher mechanisms that lead to a pro-inflammatory environment in the retina and to analyze the possible downstream effect of subretinal inflammation.
4.3.3 “Secondary inflammation” AMD Models
MC activation and subretinal MP accumulation are invariably associated with models in which the integrity of either photoreceptors or the RPE is affected. Examples include models of RD , toxic light injury, and laser-induced CNV (Karlstetter et al. 2014). In models in which the blood–retinal barrier is not clearly breached, such as RD models or light-injury models, it is sometimes assumed that the subretinal MP accumulation is constituted of MCs descending from the retina. Thisconclusion might be inaccurate, as blood-borne Mo are capable to infiltrate the retina (including the subretinal space) in the absence of a detectable breach in the blood–retinal barrier. Subretinal MPs are of both MC and Mo origin in RD models (Guo et al. 2012), photooxidative stress (Suzuki et al. 2012), and age-related accumulation in Cx3cr1-deficient mice (Sennlaub et al. 2013), with no obvious barrier breach. Bone marrow-derived MPs are also not morphologically distinguishable from MCs, best evidenced by the blood-borne microglia-like cells that replace MCs after lethal whole-body irradiation (Chen et al. 2012). MP populations infiltrating the photoreceptor cell layer and subretinal space are likely to be constituted of a mixture of MCs and iMos in the vast majority of cases. Whether an MP is of iMos or MP origin might also be more of an academic question as activated MCs could acquire a phenotype similar to iMφs. While it is yet difficult to specifically inhibit MC activation, numerous studies have analyzed the effect of iMos recruitment, in “secondary inflammation models: depletion of circulating Mo (Sakurai et al. 2003), pharmacological inhibition of Mo recruitment (Liu et al. 2013), or genetic invalidation of the CCL2/CCR2 axis (see below), to name only a few approaches. CCL2 is a major chemokine involved in the recruitment of blood-borne iMos that express CCR2 (Geissmann et al. 2010). CCL2−/− and CCR2−/− mice have been widely used in a variety of models with secondary inflammation to evaluate the role of inflammation. CCL2 expression in the retina is physiologically low/absent but CCL2 is induced in laser injury (Yamada et al. 2007), and CNV is reduced in Ccr2 −/− and Ccl2 −/− mice (Liu et al. 2013; Tsutsumi et al. 2003; Luhmann et al. 2009). Similarly, CCL2 induction and CCL2/CCR2-dependent subretinal MP accumulation are important contributing factors in photoreceptor degeneration in models of photooxidative stress (Suzuki et al. 2012; Rutar et al. 2012), in the Abca4 −/− Rdh8 −/− mouse Stargardt/AMD model (Kohno et al. 2013), the rd10 mouse (Guo et al. 2012), in the carboxyethylpyrrole immunization-induced AMD model (Cruz-Guilloty et al. 2013), and in retinal detachment (Nakazawa et al. 2007) .
< div class='tao-gold-member'>
Only gold members can continue reading. Log In or Register a > to continue
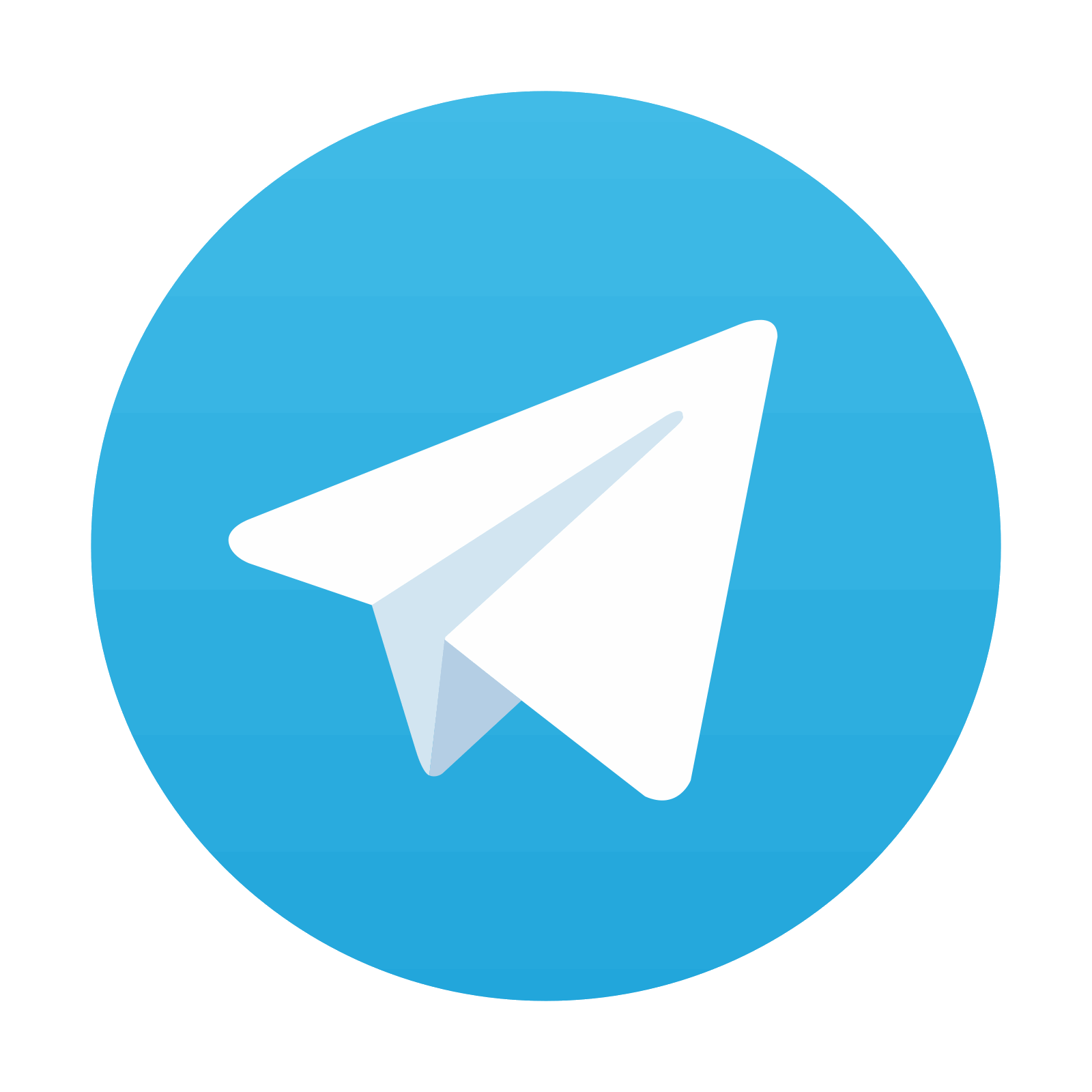
Stay updated, free articles. Join our Telegram channel
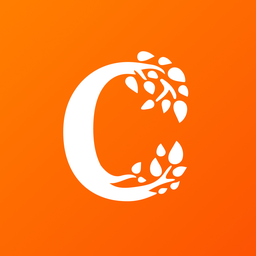
Full access? Get Clinical Tree
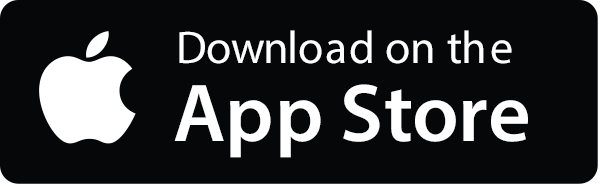
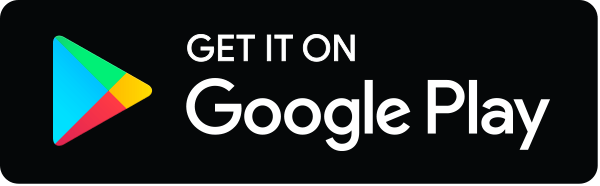