Kara Lascola and Stuart Clark‐Price Department of Clinical Sciences, College of Veterinary Medicine, Auburn University, 1220 Wire Road, Auburn, AL, 36849, USA Respiratory disease is common in horses. Infectious respiratory viruses or bacteria are easily transmitted from horse to horse, particularly with those that are regularly transported or housed in densely populated barns or pastures. As such, practitioners will be required to anesthetize horses with clinical and subclinical respiratory conditions that may impact or be impacted by general anesthesia. Additionally, as horses live longer lives because of progressive veterinary care, horses with chronic respiratory diseases such as asthma may present for anesthesia. Knowledge of the potential interaction between disease and anesthesia will improve the anesthetic care of these horses. The primary function of the respiratory system is gas exchange, specifically the diffusion of CO2 and O2 across the alveolar‐capillary membrane. Ventilatory mechanics and the anatomy of the respiratory system, including airways, pulmonary vasculature, and respiratory muscles, are specifically designed to support this function and to optimize delivery of air to the gas exchange regions of the lung. This is particularly important in the adult horse. As an athletic species, horses have relatively higher mass‐specific O2 consumption (Katz et al. 2000). At rest, minute ventilation is approximately 60–70 l/min corresponding to a minimum of 2 l/min of O2 consumed and 1.7 l/min of CO2 production. With strenuous exercise minute ventilation can increase 10‐fold (Hornicke et al. 1987; Art et al. 1990; Connally and Derksen 1994). The generation of respiratory drive and the control of breathing are primarily under central control with modulation from the cortex, central and peripheral chemoreceptors, and peripheral mechanoreceptors in response to changes in pH, PaCO2, and PaO2, respiratory disease, or other stimuli (Cunningham et al. 1986; Hazari and Farraj 2015). Neuronal networks within the brainstem provide central control of breathing and include the ventral and dorsal respiratory centers, central pattern generator, and pre‐Bötzinger complex within the medulla and the pneumotactic and apneustic centers within the pons (Garcia et al. 2011; Feldman et al. 2013; Hazari and Farraj 2015; Guyenet and Bayliss 2015; Del Negro et al. 2018; Hines 2018; Beyeler et al. 2020). These centers, particularly those in the medulla, control inspiratory drive and establish the basic rhythm of breathing as well as the volume and rate of respiration (Feldman et al. 2003; Feldman et al. 2013; Hazari and Farraj 2015). Rate and depth of respiration during automatic breathing are controlled via efferent output from the medullary respiratory center to the muscles of respiration including the diaphragm, as well as intercostal and abdominal muscles (Feldman et al. 2013; Hazari and Farraj 2015). In awake adult horses, inspiration and expiration are biphasic with each having active and passive phases (Koterba et al. 1988; Koterba et al. 1995). Modification of depth and rate of respiration in response to hypercapnia and hypoxemia are mediated by sensory afferent signals from central and peripheral sites delivered to the respiratory center (Feldman et al. 2013; Guyenet and Bayliss 2015; Prabhakar and Peng 2017; Beyeler et al. 2020). Increases in PaCO2 represent a primary stimulus for breathing. The central chemoreceptors within the ventral medulla are the most responsive to changes in PaCO2 (Hazari and Farraj 2015; Guyenet and Bayliss 2015; Beyeler et al. 2020). These receptors detect decreases in CSF and interstitial fluid pH that are consistent with increased PaCO2 and signal to the respiratory center to increase alveolar ventilation via increased inspiratory and expiratory muscle activity (Hazari and Farraj 2015; Guyenet and Bayliss 2015). Peripheral chemoreceptors include the carotid and aortic bodies which detect changes in both PaCO2 and PaO2. These receptors are most sensitive to changes in PaO2, mediating the hypoxic ventilatory drive, but will also trigger centrally mediated increases in alveolar ventilation in response to changes in pH associated with metabolic acidosis (Hazari and Farraj 2015; Prabhakar and Peng 2017). Additional sensory input to the respiratory centers comes from peripheral receptors located throughout the upper and lower respiratory tract and pulmonary tissue. Pulmonary slow adapting stretch receptors located in the walls of the bronchi and bronchioles help regulate the onset and termination of inspiration and the Hering‐Breuer Reflex in response to increases in lung volume (Coleridge and Coleridge 1986; Widdicombe 2006; Dempsey and Smith 2014; Hazari and Farraj 2015; Hines 2018). Rapidly adapting stretch (irritant) receptors are also found throughout the airways. These receptors play a minor role in modifying respiration in healthy animals but respond to mechanical and irritant stimuli from endogenous and exogenous sources (Hazari and Farraj 2015). In the upper airway, these receptors help modulate ventilation in response to pressure changes associated with airway obstruction. Stimulation of receptors in the lower airway may modify ventilation, induce bronchoconstriction, and other inflammatory responses in association with respiratory disease (Canning et al. 2006; West 2011; Hines 2018). C‐fiber receptors, when stimulated, also trigger alterations in ventilatory pattern, bronchoconstriction, and other inflammatory responses. These receptors are found within the pulmonary tissue and are stimulated in conditions of lung hyperinflation, edema, or inflammation (Hazari and Farraj 2015). Finally, carotid sinus or aortic arch baroreceptor stimulation results in hyperventilation in response to hypotension as part of the baroreceptor reflex (Hazari and Farraj 2015). The lungs of the horse are divided into the left cranial and caudal lobe and right cranial, intermediate, and caudal lobe. Horse’s lungs are poorly lobated and have incompletely developed interlobular connective tissue septa. Despite this, collateral (interlobular) ventilation is almost completely absent and, as a result, horses are less tolerant of obstructive lung disease (Robinson and Sorenson 1978). Anatomically, airway division within the lung of the horses follows the same general pattern as other species with some distinctions. The relatively straight division of right bronchus off the trachea may predispose horses to right‐sided pulmonary disease (Ainsworth and Hackett 2004) and respiratory bronchioles are poorly developed, delegating the majority of gas exchange to the alveolar‐capillary unit (McLaughlin et al. 1961; Tyler et al. 1971; Ainsworth and Hackett 2004). Similar to other athletic species, alveolar surface density is quite large in the equine lung with over 10 million alveoli and an even greater number of associated capillaries within the alveolar‐capillary network (Gehr et al. 1981). Pulmonary circulation is the predominate source of blood flow to the lung, receiving approximately 99% of cardiac output. Its primary role is delivering blood to the alveolar‐capillary network for gas exchange. In the standing horse a vertical perfusion gradient exists with blood flow favoring the ventral lung relative to the dorsal lung. Bronchial circulation accounts for only 1–2% of cardiac output and is responsible for providing O2 and nutrients to the airways and other lung structures and contributing to thermoregulation. Pulmonary lymphatic drainage is via deep and superficial lymphatics found in close approximation to the airways and in the visceral pleura, respectively (Breeze and Turk 1984). Bronchial smooth muscle activity is integral to bronchoconstriction and bronchodilation and is under control of the autonomic nervous system. Changes in airway diameter influence airway resistance and subsequently airflow to the lung. Parasympathetic input mediates smooth muscle contraction primarily via acetylcholine stimulation of M2 and M3 muscarinic receptors and resultant increases in intracellular Ca2+ concentration. In contrast, sympathetic input mediates bronchial smooth muscle relaxation primarily via stimulation of β2‐agonist receptors found in airway smooth muscle and subsequent cAMP mediated decreases in intracellular Ca2+. Other important functions under influence of the autonomic nervous system include secretion of tracheobronchial mucus, fluid transport and blood flow, immunologic regulation, and interaction with the central respiratory control networks. The mucosal lining of the trachea and bronchi consists primarily of tall columnar pseudostratified ciliated epithelium with lesser numbers of serous and goblet cells (Lopez 2001). Goblet cells and submucosal mucous glands are responsible for the production of the mucus layer. This mucus hydrates the tracheobronchial epithelial layer and is an essential component of the mucociliary apparatus involved in defense against respiratory pathogens. Immunoglobulins (IgA and IgG) as well as other immunomodulatory proteins are found in tracheal secretions. Within the bronchioles and terminal bronchioles, goblet cells and ciliated cells are gradually replaced by Clara cells which predominate within the epithelium (Lopez 2001). Clara cells produce the pulmonary epithelial lining fluid throughout this region of the lung. Cartilage within airway walls is also gradually lost (Lopez 2001) and can increase the risk of collapse of peripheral airways especially under conditions of forced expiration, such as in equine asthma. Within the alveoli, alveolar type I and type II pneumocytes predominate. Type I pneumocytes are the larger and more common cell type and are responsible for gas exchange. Although large, these cells form a very thin barrier (0.2–0.5 μm) with the capillary endothelium (Breeze and Turk 1984; West 2011). Type II pneumocytes are responsible for the production of surfactant and the replacement of damaged Type I pneumocytes. Pulmonary sur‐factant is comprised of 80–85% phospholipids and is essential for decreasing alveolar surface tension, providing alveolar stability, and maintaining gas exchange. The phospholipids within surfactant also have important immunologic functions (Christmann et al. 2006; Christmann et al. 2009). Thus, surfactant deficiency or loss of components can result in atelectasis, edema, and impaired pulmonary immunity. In addition to the mucociliary apparatus, other essential immunologic defenses within the lung include bronchus associated lymphatic tissue (BALT) and alveolar macrophages. BALT represents a network of lymphoid tissue tract that protects the respiratory tract from invading pathogens and supports alveolar macrophage function. Alveolar macrophages represent the most important component of pulmonary immunologic defense and are the predominant cell found within the alveolar lining fluid. Alveolar macrophages are the first line of defense against pathogens reaching the lower airways, possessing phagocytic, antigen presenting, and microbicidal properties. Important threats to their function include hypoxia, glucocorticoids (endogenous and exogenous), and viral infections (equine influenza virus [EIV], equine herpes virus). Pre‐anesthetic evaluation of horses with respiratory disease should begin with a standard physical examination and progress to more advanced diagnostics as warranted. Gathered information will provide a baseline for future comparison as well as guide anesthetic and therapeutic decision‐making. If the horse is suspected to have respiratory disease, viewing the horse at rest may give indication of severity. Changes in rate and character of ventilatory efforts may be seen as well as phenotypic changes, such as a “heaves line” that may indicate chronicity. Additional focus on the respiratory tract should include auscultation of all lung field as well as the cervical portion of the trachea noting the location and character of unusual findings. Use of a rebreathing technique (bag) may further amplify lung sounds and facilitate finding abnormalities, particularly in horses with a thick chest wall. Simply, a large plastic trash bag is fitted securely around the horse’s muzzle and as the horse continues to breath, increased CO2 rebreathing will result in subsequent breaths with a larger tidal volume and effort. The phase of ventilation (inspiratory versus expiratory) and the presence of stertor, stridor, crackles, wheezes, or rhonchi, or the absence of normal sounds, can help differentiate upper versus lower airway disease and help with further focused examination. Complete blood count with fibrinogen may be useful for identification of inflammatory conditions and arterial blood gas analysis can be particularly useful for characterizing conditions in which alveolar gas diffusion or ventilation are affected. Diagnostic imaging including thoracic, cervical, and head radiographs and thoracic ultrasonography are particularly useful for localizing disease to anatomical locations and for sample collection (i.e. thoracocentesis). Findings from upper airway endoscopy, including the guttural pouches, and bronchoalveolar lavage for cytology and tracheal aspirates for microbe culture, may further clarify upper versus lower airway conditions as well as help differentiate inflammatory and infectious diseases. Many of the anesthetic and analgesic medications commonly used in the anesthetic care of horses have some effect on the respiratory system. These effects can occur either locally in the respiratory tree or in lungs, systemically in the pulmonary or cardiovascular systems, or centrally in the higher centers of the central nervous system (CNS). Knowledge of the interplay between utilized drugs and the respiratory system can help identify potential adverse events that may arise in horses with specific respiratory system conditions. Drugs that may be utilized in the peri‐anesthetic period in horses that may affect the respiratory system include anticholinergics, α2‐adrenergic receptor agonists, phenothiazines, opioids, benzodiazepines, guaifenesin, dissociatives, barbiturates, propofol, alfaxalone, and volatile anesthetics. Anticholinergic drugs used in veterinary anesthesia consist mainly of atropine and glycopyrrolate and exert their action through antagonism of postganglionic muscarinic cholinergic receptors of the parasympathetic nervous system. Although not routinely used in the anesthesia of horses, the effects of this class of drugs on the respiratory system has been documented. Atropine has been used in the treatment of disorders of bronchoconstriction in the equine lung and has been shown to completely inhibit neurogenic contraction of previously contracted bronchi (Menozzi et al. 2014). Thus, atropine may be a beneficial treatment for horses where bronchoconstriction is impacting airway gas flow. However, due to the potential for adverse events (ileus, CNS toxicity, tachycardia, increased viscosity of mucous secretion, and impaired mucociliary clearance), atropine is recommended to be limited to a single rescue therapy dose for bronchodilation (Rush and Mair 2004). In anesthetized horses, bronchodilation of poorly perfused lung from anticholinergic administration may increase dead space ventilation and reduce arterial oxygen tension offsetting the benefit of bronchodilation (Lerche 2015). Xylazine is one of the most common sedative agents used in equine anesthesia; however, other α2‐adrenergic receptor agonists (detomidine, romifidine, and dexmedetomidine) are used for sedation, analgesia, and balanced anesthesia in horses. The popularity of this class of drugs is owed to the relatively rapid and reliable sedation associated with their use. However, this class of agents can have profound effects on the respiratory system. Of primary concern is the impairment of pulmonary gas exchange that can result in significant decreases of arterial oxygen and increases of arterial carbon dioxide tensions (Freeman et al. 2000; Nyman et al. 2009). This occurs from an increase in pulmonary vascular resistance and a resulting ventilation/perfusion mismatching. Xylazine, and likely other α2‐adrenergic receptor agonists, decrease respiratory rate, minute ventilation, and peak airflows after administration in horses (Raidal et al. 2017). Administration of α2‐adrenergic receptor agonists increases upper airway resistance and increases work of breathing in horses (Tomasic et al. 1997). This may be related to decreased muscle tone and collapse of the nasopharynx and oropharynx as nasotracheal or endotracheal intubation returns work of breathing to baseline effort. Acepromazine is a widely used sedative and essentially the only agent in the class of drugs that is regularly used in equine patients. Acepromazine has minimal effects on the respiratory system when used at clinically relevant doses (Steffey et al. 1985; Raidal et al. 2017). In fact, in horses experiencing pulmonary ventilation/perfusion mismatch after sedation with an α2‐adrenergic receptor agonists, administration of acepromazine may reduce the fall in arterial oxygen tension (Marntell et al. 2005). This is thought to occur due to the ability of acepromazine to counteract the vasoconstrictive effects of the α2‐adrenergic receptor agonists through blockade of vascular α1‐adrenergic receptors. Acepromazine appears to have no effect on bronchial diameter but can cause pronounced muscle relaxation which may be problematic in horses with respiratory muscle fatigue. The use of opioids in horses, particularly full μ‐agonists, continues to be debated due to questions on analgesic efficacy. However, the respiratory depressant effects of opioids are well recognized in veterinary species. In awake horses, the respiratory depression associated with opioids is generally not problematic and usually does not require intervention (Muir et al. 1978; Robertson et al. 1981). However, in anesthetized animals, respiratory depression manifests as decreased minute ventilation with a concomitant rise in arterial carbon dioxide tension. Subsequently, arterial pH drops below optimal and acidemia ensues. At lower doses of opioids, the respiratory rhythm is disturbed, characterized as a slower rate and increased inspiratory periods. As opioid doses increase, tidal volume becomes reduced due to decreased inputs from opioid sensitive chemoreceptors. Interestingly, this respiratory pattern has been described as “quantal” due to the nature in which action potentials are not transmitter further in the respiratory centers similar to Mobitz type‐II second‐degree heart block (Pattinson 2008). The effects of opioids on the respiratory system are not limited to central control of ventilation. Opioids can exert effects locally on the lungs including generation of pulmonary edema through endothelial dysfunction, dose‐related bronchoconstriction, and immunosuppression of host cellular and innate defenses (Yamanaka and Sadikot 2013). Benzodiazepines are frequently used in the anesthesia of horse for combination with other medications for muscle relaxing effects. On their own, benzodiazepines, at clinically relevant doses, have negligible effects on the respiratory system. Similar to benzodiazepines, guaifenesin is used in combination with other anesthetic drugs to provide muscle relaxation. Useful for total intravenous anesthesia with ketamine and xylazine, respiratory depression from this combination can occur resulting in hypoventilation and hypoxemia (Greene et al. 1986). Ketamine is one of the most commonly used anesthetic induction agents and intravenous maintenance agents in horses. It is also used as an anesthetic adjunctive agent during inhalant anesthesia either as bolus injections or a continuous infusion. Thus, the effects of this class of drugs on the equine respiratory system have been studied. In general ketamine does not cause respiratory depression, in fact, appropriate responses to hypoxemia and increased carbon dioxide are maintained during ketamine anesthesia (Soliman et al. 1975). However, respiratory patterns do change after ketamine administration and are frequently described as an “apneustic” pattern. This is characterized as a breath‐holding pattern during inspiration and a relatively quick expiration immediately followed by a prolonged inspiration again (Jaspar et al. 1983). Ketamine has also been shown to be a potent bronchodilator. The use of ketamine in patients with status asthmaticus showed improved outcomes in patients with asthma unresponsive to conventional treatments (Goyal and Agrawal 2013). Ketamine may be a preferred agent in horses with bronchial obstructive diseases such as equine asthma. Barbiturates have been utilized as anesthesia induction and maintenance agents for veterinary patients since at least the 1940s and thiopental is probably the more favored barbiturate agent for equine induction. In general, barbiturates can have profound dose‐dependent depressant effects on the respiratory system. Direct depression of the ventilatory centers occur after administration and animals have decreased responsiveness to both hypoxemia and hypercapnia (Tyagi et al. 1964; Hirshman et al. 1975; Quandt et al. 1998). Propofol as a sole induction agent in adult and/or large horses is problematic due to the large volumes necessary; however, it can be useful in smaller horses and foals and when combined with other agents such as ketamine (Mama et al. 1995). The effects of propofol on the respiratory system are very similar to the barbiturates in that direct respiratory depression occurs as well as sensitivity to changes in arterial oxygen and carbon dioxide tensions. These effects appear to be both dose and rate of administration dependent. Decreases in tidal volume and respiratory rate can be expected and supplemental oxygen and ventilatory support should be made available when propofol is used (Mama et al. 1995; Quandt et al. 1998). Alfaxalone is one of the newest induction agents available in veterinary medicine, and limited comparative data is available with regard to its use in horses. The depressant effects of alfaxalone on the respiratory system are expected to be similar to or slightly less than propofol. When using alfaxalone in horses, there should be an expectation of clinically relevant respiratory depression, and options for ventilatory support should be available (Ohmura et al. 2016). Clinically useful volatile anesthetics in veterinary medicine include isoflurane and sevoflurane with halothane continuing to be utilized in some countries. Volatile anesthetics have various effects on the respiratory system that are widely considered dose dependent and to some degree, species dependent. Horses in particular can have profound changes in respiratory physiology and function related to volatile anesthetic exposure due to the complex nature and anatomy of the equine lung and recumbency during anesthesia (Auckburally and Nyman 2017). As dose of volatile anesthetics are increased (i.e. increased minimum alveolar concentration or MAC), respiratory rate and tidal volume decrease to the point where ventilation ceases and death ensues without intervention. Additionally, central and peripheral chemoreceptors become less sensitive to carbon dioxide tension further decreasing the drive to ventilate. Hypoxic pulmonary vasoconstriction is an important component to maintaining ventilation/perfusion matching in the equine lung. All modern volatile anesthetics inhibit hypoxic pulmonary vasoconstriction in a dose‐dependent manner (Lumb and Slinger 2015). This can lead to a significant amount of blood perfusion to underventilated lung. Blood flow returning to the pulmonary circulation from this area has not had the opportunity to become oxygenated and, when combining with oxygenated blood, can result in a hypoxic mixture. Horses tend to suffer from hypoxemia during anesthesia at a higher rate than most veterinary species, and techniques that reduce the amount of inhalant anesthetic utilized may improve oxygenation (Auckburally and Nyman 2017). Interestingly, injectable anesthetics do not appear to affect hypoxic pulmonary vasoconstriction (Lumb and Slinger 2015). General considerations for vital signs monitoring and support of anesthetized horses can be found elsewhere but should be in place as a starting point when anesthetizing horses with respiratory diseases. Horses with specific respiratory tract conditions (discussed blow) may require more intensive monitoring and support of gas exchange and diffusion or ventilation of alveoli. In general, upper airway diseases and conditions are more commonly associated with obstruction of airflow while lower airway diseases and conditions are more commonly associated with impaired gas exchange and diffusion. Airway management for upper airway conditions is focused on minimizing resistance to airflow and may include alternatives to orotracheal intubation including nasotracheal intubation or intubation though a tracheotomy (Figure 3.1). Management for lower airway conditions may include application of specific ventilator strategies or use of aerosolized medications to improve oxygenation. Use of pulse oximetry, capnography, and arterial blood gas analysis can be useful to determine when intervention is necessary and how effective intervention strategies are. Pulse oximetry can be helpful for determining oxygen content in the arterial blood of a horse before, during, and after anesthesia (recovery) and can be used to indicate need for supplemental oxygen. Caution must be used when interpreting a pulse oximeter reading as skin thickness and pigmentation in horse skin may interfere with the reading. An arterial blood gas may be necessary for verification. Placement of an arterial catheter for direct blood pressure measurements facilitates collection of arterial blood for gas analysis. However, it is frequently not practical to place the catheter in an awake horse; therefore, direct puncture of an artery may be necessary for sample collection. Sites for sample collection in the awake horse include the various branched of the facial artery with the branch palpated just caudal and ventral to the ventral aspect of the rim of the orbit being most convenient. Arterial blood gas analysis for PaO2 and PaCO2 are the most important variable for assessing respiratory function. Venous blood gas analysis may be useful for PvO2 and lactate concentrations and can be acquired from the jugular vein or transverse facial venous sinus (Lascola et al. 2017). Respiratory function can also be monitored with capnometry. Sampling of airway gases during anesthesia can provide graphical representation of CO2 during inspiration and expiration. This graph (capnography) can be analyzed for airway status and spontaneous or mechanical ventilatory function. Real‐time information on airway obstruction, hypoventilation, hyperventilation, rebreathing, and anesthesia breathing circuit integrity are some examples of available information. Other methods of respiratory and ventilatory monitoring of horses include spirometry and electrical impedance tomography, although this technology may not be practical for routine clinical use (Moens 2013; Auer et al. 2019). Detailed review information on respiratory monitoring in anesthetized horses can be found in previous publications (Hubbell and Muir 2009; Moens 2013). Additionally, information on the use and interpretation of capnography can be found at www.capnograph.com. Figure 3.1 Intubation of an anesthetized horse through a tracheotomy due to inability to pass an orotracheal tube. The horse is being prepared for surgical drainage of the guttural pouch. During the induction process, an amount of time will elapse between a horse becoming recumbent and intubation and initiation of oxygen and inhalant administration from an anesthesia machine. During this period, secondary to recumbency and the effect of anesthetic drugs, a decrease in PaO2 may occur (van Oostrom et al. 2015). In horses with respiratory diseases that are associated with hypoxemia, a decrease in PaO2 during induction may result in a critical hypoxemia that could affect health status. Placement of an intranasal cannula to the level of the medial canthus of the eye and provision of oxygen at 15 l/min for 3 minutes prior to induction may maintain PaO2 above concentrations considered to indicate hypoxemia (van Oostrom et al. 2015). This may allow for a safer transition of horses from induction to maintenance with inhalant delivered in oxygen. Mechanical ventilation (intermittent positive pressure ventilation) is frequently required for anesthetized adult horses. Moreover, horses with respiratory diseases, particularly diseases of the lower respiratory tract, may benefit from mechanical ventilation initiated shortly after induction. In fact, horses that are placed on mechanical ventilation immediately after induction of anesthesia maintain more normal PaCO2, pH, and PaO2 compared to spontaneously breathing horses or those that are allowed to initially spontaneously breathe and then be subsequently mechanically ventilated (Day et al. 1995; Kerr and McDonell 2009). Ventilation techniques for improvement in ventilatory variables, particularly PaO2, are widely reported and include the use of continuous positive airway pressure (CPAP), recruitment maneuvers, and use of various amounts of positive end‐expiratory pressure (PEEP) (Hopster et al. 2011; Mosing et al. 2013; Andrade et al. 2019). Description and direction on the use of each ventilatory technique is beyond the scope of this chapter, and the reader is directed to the references for further reading. Figure 3.2 A wye piece with a fitting for administration of albuterol into the anesthetic breathing circuit. Beyond mechanical ventilation, inhaled medications may be useful for improving PaO2 in anesthetized horses. In hypoxemic horses, albuterol (2 μg/kg) administered via inhalation can increase PaO2 (Robertson and Bailey 2002). Administration of each actuation or “puff” must coincide with the beginning of the inhalation cycle of mechanical ventilation or a spontaneous breath and adaptation of a wye piece with a port facilitates administration (Figure 3.2). The improvement of PaO2 may be the result of bronchodilation and improved aeration of alveoli or increased cardiac output to ventilated lung. Pulsed administration of inhaled nitric oxide can similarly improve PaO2 in anesthetized horses. Although specialized equipment is necessary to administer nitric oxide into a breathing circuit, PaO2 increases via reduction in the amount of blood shunted away from ventilated alveoli thus increasing the amount of blood available for gas exchange (Wiklund et al. 2020). The recovery period has the potential to be one of the most complicated areas of anesthesia of horses with respiratory diseases. Once placed in a recovery box, respiratory support and monitoring become challenging. The goal for recovery is a move to a standing position that is free from excitement and delirium which may exacerbate respiratory compromise. While continuation of mechanical ventilation is not feasible, assisted ventilation with a demand valve prior to extubation can provide continued oxygen support (Figure 3.3). Once extubated, nasal insufflation with oxygen (15 l/min) can be utilized for oxygen support. For horses with a tracheostomy, a self‐retaining tracheotomy can be placed and an oxygen line can be inserted through it directly into the trachea (Figure 3.4). Acepromazine can be used to reduce anxiety during recovery and improve arterial oxygenation (Marntell et al. 2005). If alpha‐2 agonist drugs are used for sedation during recovery, the addition of acepromazine may counteract the vascular effect that can exacerbate ventilation to perfusion mismatching associated with alpha‐2 agonists (Marntell and Nyman 1996; Nyman et al. 2009). Figure 3.3 Use of a demand valve to support ventilation of a horse in a recovery stall. Figure 3.4 Insufflation of oxygen though a self‐retaining tracheostomy in a horse recovering from anesthesia. While information on the pathophysiology and treatment of inflammatory and infectious diseases are well known, there is minimal published research data on anesthetic techniques for horses with respiratory diseases. Much of the information in this chapter regarding anesthetic techniques is derived from data in other species including humans, and clinical experience and anecdotal information from the veterinary anesthesia community. Streptococcus equi subsp. equi (Equine Strangles) is one of the most common infectious upper respiratory tract diseases in horses and is reportable within the United States. S. equi is a gram‐positive β‐hemolytic coccoid bacterium and, unlike S. equi subsp. zooepidemicus, is a primary pathogen in horses, donkeys, and mules. Clinical disease is most common in horses one to six years of age, but can also develop in older horses and foals (Duffee et al. 2015). S. equi is highly infectious with morbidity reaching 100% in naïve horses. Following infection, protective immunity develops in approximately 75% of horses and persists for approximately five years (Hamlen et al. 1994; Boyle et al. 2018). Transmission of S. equi is via direct contact with infected horses or contaminated fomites. Horses with obvious signs of clinical disease, asymptomatic carriers, and horses recovering from infection but no longer demonstrating clinical signs all contribute to disease outbreaks and persistence of the organism within a population. Chronic carriers continue to shed S. equi for more than six weeks and sometimes for months‐to‐years after infection (Mallicote 2015). Carrier prevalence has been reported to be between 10% and 40% in naturally infected horses (Newton et al. 2000; Duffee et al. 2015; Boyle et al. 2018). In a recent report (Duffee et al. 2015), approximately 30% of horses diagnosed with strangles via upper airway endoscopy did not present with typical clinical signs of disease suggesting that acutely infected horses may also serve as silent shedders. The pathogenesis of strangles has been well described (Timoney and Kumar 2008; Waller 2014; Mallicote 2015; Boyle et al. 2018). Bacteria gain entry to the upper respiratory tract primarily via inhalation, adhere to the mucosal surface of pharyngeal tonsillar tissue, rapidly translocate to deeper tonsillar tissues, and subsequently colonize regional lymph nodes within a few hours of infection. Bacterial replication and neutrophil influx with subsequent lymphadenopathy and abscessation characterize clinical strangles. Spread of S. equi beyond the upper respiratory via lymphatic or hematogenous routes is responsible for metastatic strangles and abscess formation elsewhere in the body (Mallicote 2015; Boyle et al. 2018). Bacterial virulence factors contribute to S. equi pathogenicity and evasion of host defenses. These factors include surface proteins such as the Strep equi M‐like (SeM) protein, antiphagocytic proteins, and several bacterial toxins (Timoney et al. 2014; Boyle et al. 2018). The SeM protein not only plays a central role in virulence but the identification of SeM protein DNA or antibodies is also used to diagnose strangles. Potential complications associated with S. equi infection are numerous. The most frequently reported include airway obstruction, guttural pouch empyema and chondroid formation, metastatic abscesses, and the immunologic sequelae of purpura hemorrhagica and S. equi–associated myositis (Mallicote 2015; Boyle et al. 2018). Airway obstruction most often occurs with pharyngeal compression secondary to lymphadenopathy and abscessation of the retropharyngeal lymph nodes. Tracheal compression from abscesses located in the cranial mediastinum or at the thoracic inlet has been reported more rarely. Metastatic S. equi can involve lymph nodes and organ systems throughout the body including the CNS, abdomen, lungs, and other thoracic cavity structures (Duffee et al. 2015; Boyle et al. 2018). Empyema of the guttural pouch is caused by accumulation within the pouch of purulent material, debris, and exudate associated with bacterial infection (Judy et al. 1999; Freeman 2015). Strangles represents the most significant cause of empyema and involves rupture and drainage of a retropharyngeal lymph node abscess into the floor of the pouch. Less common causes of empyema include other bacterial infections of the upper respiratory tract (especially S. equi subsp. zooepidemicus), and, more rarely, trauma, stenosis of the pharyngeal orifice, or infusion of irritating substances into the pouch (Freeman 2015). In horses with strangles, empyema is typically bilateral, with unilateral disease more common with other causes (Sweeney et al. 1987; Pascoe 2019). Purulent material can become inspissated resulting in chondroid formation and the development of a S. equi carrier state in horses with chronic empyema. Characteristic signs of strangles become evident 2–14 days after infection and include pyrexia (≥103 °F), lethargy, serous progressing to mucopurulent nasal discharge, and lymphadenopathy with lymph node abscessation of the submandibular and/or retropharyngeal lymph nodes (Boyle et al. 2018). Pyrexia, usually the first clinical sign noted, may not always be identified in horses with clinical strangles (Duffee et al. 2015). In most horses, bacterial shedding begins within 2–3 days of fever and continues for a minimum of 2 weeks. Maturation and rupture of abscesses occurs in 7–14 days after the onset of clinical signs. Specific signs associated with metastatic strangles typically correspond to the organ system involved. Clinical presentation suggesting empyema include mucopurulent nasal discharge that is most prominent with the head lowered, swelling over the parotid region, cough, upper respiratory noise, and occasionally dyspnea (Judy et al. 1999). Inflammation or irritation of the cranial nerves within the guttural pouches may also cause dysphagia or other neuropathies. Because of the highly infectious nature of S equi, timely and accurate diagnosis of strangles is critical. Horses with confirmed or suspected S. equi infection should be isolated and placed under strict biosecurity protocols until cleared of infection. In many states, diagnostic protocols and quarantine regulations are under the direction of the state veterinarian. In animals with overt clinical signs, direct sampling and culture of abscess or purulent exudate is ideal but not always possible. Culture or polymerase chain reaction (PCR) to detect SeM protein DNA can also be performed on guttural pouch (preferred) or nasopharyngeal washes. Serologic testing of SeM antibodies can be useful in horses suspected of complications associated with S. equi infection (Boyle et al. 2009). Hematologic findings are typically nonspecific and indicative of an inflammatory process. Endoscopic evaluation of the upper airways and guttural pouches is useful for evaluating horses with strangles, and can provide a definitive diagnosis when guttural pouch empyema is suspected. Both pouches should be evaluated, especially in horses with confirmed or suspected equine strangles infection. Exudate should be collected and submitted for microbial culture and PCR testing. Radiography can be useful to identify fluid lines within the pouches when endoscopy is not available (Figure 3.5). Additional diagnostics such as ultrasound, or other advanced imaging, may be warranted in horses with metastatic strangles, depending on body systems involved. Figure 3.5 A lateral radiograph of a horse with guttural pouch empyema. The black arrows indicate a fluid line in the guttural pouch. Treatment of horses with strangles depends on the stage and manifestation of disease. In horses with uncomplicated strangles, treatment is primarily supportive and the prognosis for full recovery is generally very good. Administration of antimicrobials remains controversial as it may prolong the course of disease or interfere with the development of lasting immunity. For this reason, antimicrobial use is generally restricted to treating horses with complications associated with S. equi infection such as dyspnea or significant systemic inflammation, and when metastatic strangles, purpura hemorrhagica, or S. equi associated myositis are identified. Horses with dyspnea associated with pharyngeal compression may require tracheostomy and in rare cases, intensive supportive care (intravenous fluids, placement of indwelling feeding tube, antimicrobial drugs) is warranted. Surgical removal or drainage of abscesses may be performed in horses with metastatic strangles. In horses with guttural pouch empyema, specific medical management involves removal of exudate through repeated guttural pouch lavage using polyionic or other non‐irritating solution. Endoscopic guided removal of chondroids can be attempted (Freeman and Hardy 2012). Surgical drainage of affected pouches is reserved for severe or chronic cases nonresponsive to lavage, cases with numerous chondroids, or cases where stenosis of the pharyngeal orifice is identified (Freeman 2015). Local or systemic antimicrobial therapy is controversial, especially in horses with strangles (Boyle et al. 2018), but may be useful in select cases with accompanying microbial culture. Additional therapy may be needed in horses with dysphagia, or other neurologic deficits. The vast majority of horses with strangles will not require surgical intervention, thus anesthesia is usually not necessary. However, horses with certain complications previously described may require anesthesia or sedation for tracheostomy or surgical approach and drainage of internal abscesses. When formulating an anesthetic plan, minimization of contamination of equipment and surrounding spaces is paramount to prevent infection of subsequently anesthetized horses. Use of personal protective equipment including facemasks and gloves should be routine as Streptococcus equi subsp. equi has the potential for zoonotic infection (Baracco 2019). Biosecurity includes isolation of infected or suspected infected horses, use of footbaths and protective clothing and deep cleaning any area that may have had exposure. Streptococcus equi subsp. equi is susceptible to most standard disinfectants (diluted bleach or quaternary ammonium compounds); however all organic debris must be diligently removed as bacteria can survive for as long as three days (Mallicote 2015). For surgical removal of chondroids or drainage of guttural pouch empyema, surgery can be performed in the standing horse facilitated by sedation and local anesthesia (Perkins et al. 2006). Sedation can be performed with xylazine or detomidine with butorphanol added for additional analgesia/sedation. After preparing the area for surgery, 20–30 ml of local anesthetic can be infused directly into the tissue, and an approach can be made via the modified Whitehouse approach to the guttural pouch. For deeper abscess in other areas of the body or large numbers of chondroids within the guttural pouches, general anesthesia may be necessary (Furniss et al. 2007). During recovery of horses that have had diseases of the guttural pouch, neurologic complication such as dysphagia, abnormal soft palate positioning, laryngeal paralysis, and Horner syndrome can occur (Borges and Watanabe 2011). Airway obstruction may occur, and horses should be monitored closely as re‐intubation or tracheostomy may be required to re‐establish patency. The guttural pouches are anatomical structures that are unique to equids and a few other species and whose exact function is unknown (Baptiste et al. 2000). They represent paired diverticula of the auditory tubes that are located anatomically in the retropharyngeal space and that communicate with the pharynx through slit‐like openings (Freeman and Hardy 2012; Pascoe 2019). Each pouch has a capacity of approximately 300 ml in the adult horse, but can distend to accommodate larger volumes of air or fluid. Each guttural pouch is divided into a larger medial and smaller lateral compartment by the stylohyoid bone. The mucosal lining consists of ciliated pseudostratified epithelium, goblet cells, and subepithelial aggregates of lymphocytic tissue. Several important structures within or in close proximation to the guttural pouch can become damaged in infectious or inflammatory conditions such as guttural pouch empyema or mycosis. Within the medial compartment, neural and vascular structures include cranial nerves IX, X, XI, XII, the cranial cervical ganglion, the cervical sympathetic trunk, and internal carotid artery. The pharyngeal branch of the vagus (CN X), the cranial laryngeal nerve, and the retropharyngeal lymph nodes are found ventral to the floor of the medial compartment. Within the lateral compartment neural and vascular structures include the facial (CN VII) and chorda tympani nerves, branches of the mandibular nerve, the external carotid and maxillary arteries, and the maxillary vein. The most significant medical disorders of the guttural pouches include guttural pouch empyema (see Section on Equine Strangles) and guttural pouch mycosis. Guttural pouch mycosis is defined as fungal invasion of the mucosal lining within one or both guttural pouches (Freeman 2015; Pascoe 2019). While epistaxis represents the most common clinical sign associated with this condition (Freeman 2015), the clinical manifestation of this condition varies among affected horses depending on the severity and location of fungal invasion and the specific involvement of underlying neuro‐ vascular structures. The etiopathogenesis of guttural pouch mycosis remains incompletely defined (Pascoe 2019). Age, breed, and sex predilections are not recognized among affected horses (Freeman 2015). The condition does appear to be more common in stabled horses residing in the northern hemisphere and during warmer seasons (Freeman 2015). While Aspergillus species (A. fumigatus, A. nidulans) are most frequently isolated (Freeman 2015; Pascoe 2019), other fungal species can be identified. Fungal plaques can develop anywhere within the guttural pouch. One of the more common locations for plaque formation is dorsally in the medial compartment in association with the internal carotid artery (Cook 1968; Freeman 2015; Pascoe 2019). Plaques can involve additional neuro‐vascular or other structures anywhere within the guttural pouch such as the maxillary artery, cranial nerves, muscle, or bone. Fungal plaques attach to the underlying tissue through a diphtheritic membrane within which necrotic tissue and numerous bacterial and fungal organisms can be found (Freeman 2015; Pascoe 2019). These plaques cause significant inflammation, erosion, and necrosis of underlying tissue which can ultimately result in hemorrhage, neuropathy, or other tissue damage (Cook 1968). Specific clinical signs of guttural pouch mycosis correspond to the location of fungal invasion. Epistaxis is most common (Freeman 2015) and may start as mild and intermittent and progressing over time, or can present acutely with severe‐to‐fatal hemorrhage. After epistaxis, dysphagia represents the second most common clinical abnormality and develops when there is involvement of cranial nerves IX, X, and XII (Cook 1968; Freeman 2015; Pascoe 2019). Dysphagic horses typically present with nasal discharge that contains feed or saliva and may become mucopurulent. Cough while eating and tongue paralysis may be noted. Other less common clinical presentations associated with cranial nerve neuropathy may include Horner’s syndrome, respiratory noise secondary to recurrent laryngeal neuropathy, and signs of facial nerve paralysis (ear droop, muzzle deviation) (Cook 1968; Cook et al. 1968; Freeman and Hardy 2012). Horses may appear painful when palpated over the parotid region and may present with abnormal head posture or demonstrate head or neck extension. Clinical signs of epistaxis or dysphagia should warrant inclusion of guttural pouch mycosis as a differential. Endoscopy of both guttural pouches is performed to confirm the diagnosis (Lepage and Piccot‐Crézollet 2005). Fungal plaques vary in color and may appear as discrete lesions or as diffusely distributed patches (Cook 1968; Cook et al. 1968; Pascoe 2019
3
Anesthetic Management for Inflammatory or Infectious Respiratory Diseases
Introduction
Respiratory Physiology
General Considerations for Anesthesia of Horses with Inflammatory/Infection Respiratory Disease
Pre‐anesthetic Evaluation
Anesthetic, Analgesic, and Adjunctive Drugs
Anticholinergics
α2‐Adrenergic Receptor Agonists
Phenothiazines
Opioids
Benzodiazepines
Guaifenesin
Dissociatives
Barbiturates
Propofol
Alfaxalone
Volatile Anesthetics
Monitoring and Support During Anesthesia
Recovery
Anesthetic Consideration of Horses with Specific Infectious or Inflammatory Diseases of the Upper Respiratory System
Streptococcus equi subsp. equi (Equine Strangles)
Anesthetic Considerations
Disorders of the Guttural Pouch
Guttural Pouch Anatomy
Guttural Pouch Mycosis
Pathophysiology
Stay updated, free articles. Join our Telegram channel
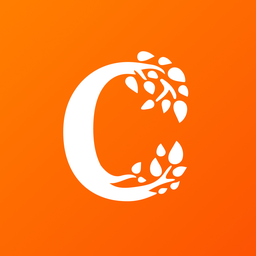
Full access? Get Clinical Tree
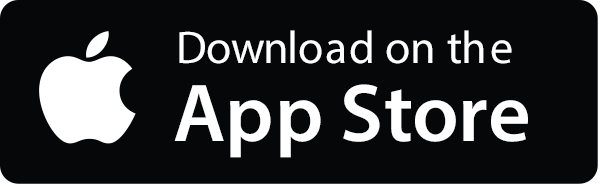
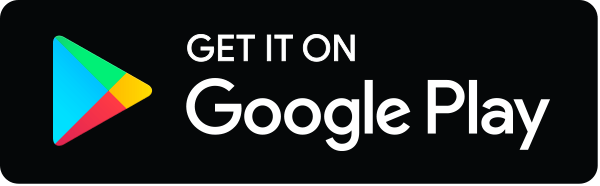