CHAPTER 2 Cellular and molecular mechanisms in embryonic development
INITIAL EMBRYONIC DEVELOPMENT AND GESTATIONAL PERIODS
Embryonic development encompasses all the processes whereby a single cell – the fertilized egg or zygote – gives rise to first an embryo, then a fetus which at birth has the capacity to adapt to post-natal life. These processes occur as a continuum but, for convenience, they may be arbitrarily divided into successive periods. Thus, intra-uterine development is often divided into an embryonic period, where all major organ systems are established, and a fetal period, which consists primarily of growth and organ refinement (see Chapter 18). Development of the organism does not stop with birth, however; organs continue to grow and mature at least until puberty and many tissues need continuous replenishment throughout life. Aging and death may therefore also be included the natural developmental process of the organism.
The embryonic period is initiated at fertilization when the oocyte, covered by the zona pellucida, is penetrated by the fertilizing spermatozoon resulting in the formation of the one-celled zygote, in which a maternal pronucleus (from the oocyte) and a paternal pronucleus (from the spermatozoon) develop (Fig. 2-1; see Chapter 5). At the first post-fertilization mitosis the zygote develops into the 2-cell embryo. These two, and subsequent early embryonic cells, are for a period referred to as blastomeres. During the initial mitotic divisions of blastomeres, the increase in the number is not accompanied by any increase in the volume; the overall volume of the embryo remains constant as the size of the blastomeres halves with each division. Such unique cell divisions are referred to as cleavages (Chapter 6). Then, coincident with the cells having reached a certain minimal size through cleavage divisions, a phase of cell growth is introduced into the cell cycles, with daughter cells growing to approximately the size of the mother cell. This more constant cell size, together with abundant cell proliferation and deposition of extra-cellular material, then leads to an increase in the size of the embryo as a whole. Both cell proliferation and cell growth are principles of general cell biology and will not be dealt with in further detail in this book.
The blastomeres eventually form a small mulberry-like cluster of cells referred to as the morula (Fig. 2-1; see Chapter 6). Initially, the morula is characterized by bulging individual blastomeres; later, outer cells will adhere tightly to each other and form a more uniform surface of the morula. This process is referred to as compaction. The outer cells develop into the trophectoderm. Subsequently, during the process of blastulation, a fluid-filled cavity, the blastocyst cavity, develops inside the trophectoderm, and the inner cells, forming the inner cell mass (ICM), gather at one pole of the embryo which is now known as a blastocyst. The trophectoderm will participate in placenta formation (see Chapter 9) while the ICM gives rise to the embryo proper. The blastocyst expands, hatches from the zona pellucida, and later, towards the end of blastulation, the ICM forms an internal and external cell layer, referred to as the hypoblast and epiblast, respectively, to establish the bilaminar embryonic disc. Formation of the disc is, in domestic animal species, associated with the removal of the trophectoderm covering the epiblast (see Chapter 6). Along with this, the overall shape of the embryo changes from spherical to ovoid and further to tubular and filamentous except in the horse. The time period from fertilization to completion of blastulation lasts about 10 to12 days in pigs, sheep, goat, and cat, 14 days in cattle and horses, and 16 days in the dog.
During the subsequent phase of the embryonic period, the bilaminar embryonic disc is transformed into a trilaminar embryonic disc, through the process of gastrulation (Fig. 2-1; see Chapter 7). Gastrulation leads to formation of the three somatic germ layers, ectoderm, mesoderm and endoderm, and formation of the primordial germ cells, the progenitors of the germ cell lineage. Early during the process of gastrulation, a portion of the trophectoderm located around the embryonic disc, together with its underlying extra-embryonic mesoderm, forms amniotic folds that finally fuse and enclose the disc in the amniotic cavity.
Following gastrulation, the three somatic germ layers further differentiate into various cell types and finally form the outline of most organ systems, thereby defining the end of the embryonic period (Fig. 2-2). The increasing number of cell types derived from the initial three somatic cell lineages formed during the process of gastrulation obviously requires intricate regulation of cell behaviour and organization of cells in order to create a complete organism with its many different, but interdependent, tissues and organs. Different mechanisms regulating embryonic development should not be looked at in isolation but, again for convenience, the concomitant processes of differentiation, patterning and morphogenesis will be outlined separately below.
The embryonic period is followed by the fetal period, lasting until term, with growth, maturation and remodelling of the organ systems. The development of each organ system, collectively referred to as ‘special embryology’, will be covered in subsequent chapters. In general, most embryonic loss occurs early in the embryonic period. This is also the time when the embryo is most susceptible to teratogens (see Chapter 19).
DIFFERENTIATION
Cell differentiation is ultimately regulated through differential gene expression. Like a stream running down the side of a mountain with its flow branching many times before it reaches the bottom, so do embryonic cells differentiate from common origins to gradually form specialized cell types (Figs. 1-4, 2-2). And just as a leaf dropped onto the stream follows one path only, so does a particular cell follow a single line of differentiation. However, for both the leaf and the cell, numerous decisions are taken along the way to the final destination. Hence, cell differentiation during embryonic development involves many branch points where lineages divide and sequential decisions on differentiation are taken. As outlined above, these decisions are at first reversible (cell specification), but later become irreversible (cell determination).
Induction
The first embryologist to investigate induction was Hans Spemann (see Chapter 1). In amphibian embryos, he noted that lens formation in the surface ectoderm was achieved only when a close interaction between the optic vesicle from the developing brain and the surface ectoderm was allowed to occur (Fig. 2-3; see Chapter 11). Spemann was able to remove the optic vesicle before this interaction occurred and show that, as a consequence, no lens-formation was seen in the overlying ectoderm. Interestingly, if the optic vesicle was placed beneath the trunk surface ectoderm, it was not possible to induce lens formation in the overlying ectoderm. In other words, the trunk surface ectoderm was not competent for lens induction.
Another example of induction is the generation of the nervous system through the induction of neuroectoderm (Fig. 2-4, see Chapter 8). Early ectodermal cells may either differentiate into surface ectoderm (and thence the epidermis), or neuroectoderm (thence forming the nervous system). Initially, early ectodermal cells are uncommitted (or naïve) and competent to differentiate in either direction. However, signals from the notochord (an important midline structure in vertebrate species appearing during gastrulation; see Chapter 7) induce overlying ectodermal cells to differentiate into neuroectoderm. In particular the signalling molecule known as Sonic hedgehog (Shh) is pivotal in this process. Ectodermal cells outside the embryonic midline receive no inductive signals and form surface ectoderm by default. Experiments have shown that: if the notochord is removed, only surface ectoderm will form; placing notochordal tissue outside the embryonic midline will induce the formation of neuroectoderm instead of surface ectoderm; and midline ectoderm removed from the influence of underlying notochord will form surface ectoderm. This example illustrates the fact that during cell specification the fate of cell differentiation can be altered by changing the position of the cell within the embryo. Once cell determination has occurred, this plasticity is lost.
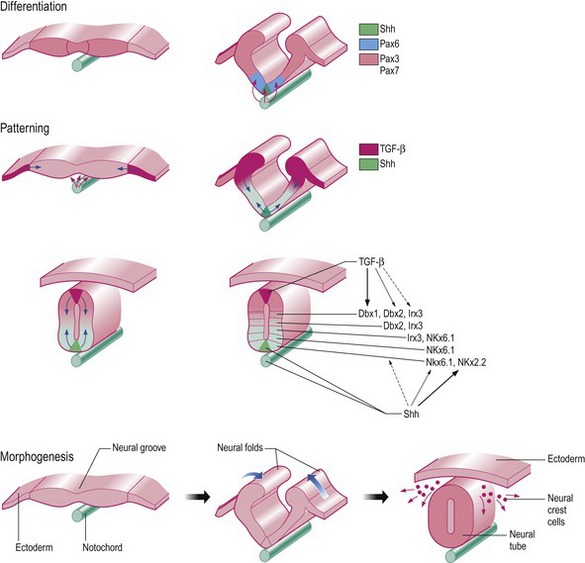
Fig. 2-4: The initial development of the nervous system as an example of differentiation, patterning, and morphogenesis. Differentiation: Sonic hedgehog (Shh) secreted from the notochord induces formation of the overlying neuroectoderm. Already during induction, a gradient of this molecule is involved in determining the structure of the neural tube by a rough patterning into dorso-ventral zones by expression of the transcription factors Pax 6 (ventrally) and Pax 3 and 7 (dorsally). Patterning: Shh from the notochord (as already mentioned) and TGF-β from the surface ectoderm is involved in the rough dorso-ventral patterning of the neural tube resulting in further generations of transcription factors controlling the finer patterning of different layers of afferent (dorsal) and efferent (ventral) neurons. Morphogenesis: The change in shape from the flat neural plate into a neural tube is caused by the formation of hinge points where cells become apically constricted and proliferation of the surface ectoderm at the margins of the neural plate pushes the sides together. Neural crest cells leave the neural tube at the hinge point to form other structures.
Modified from Strachan and Read (2004).
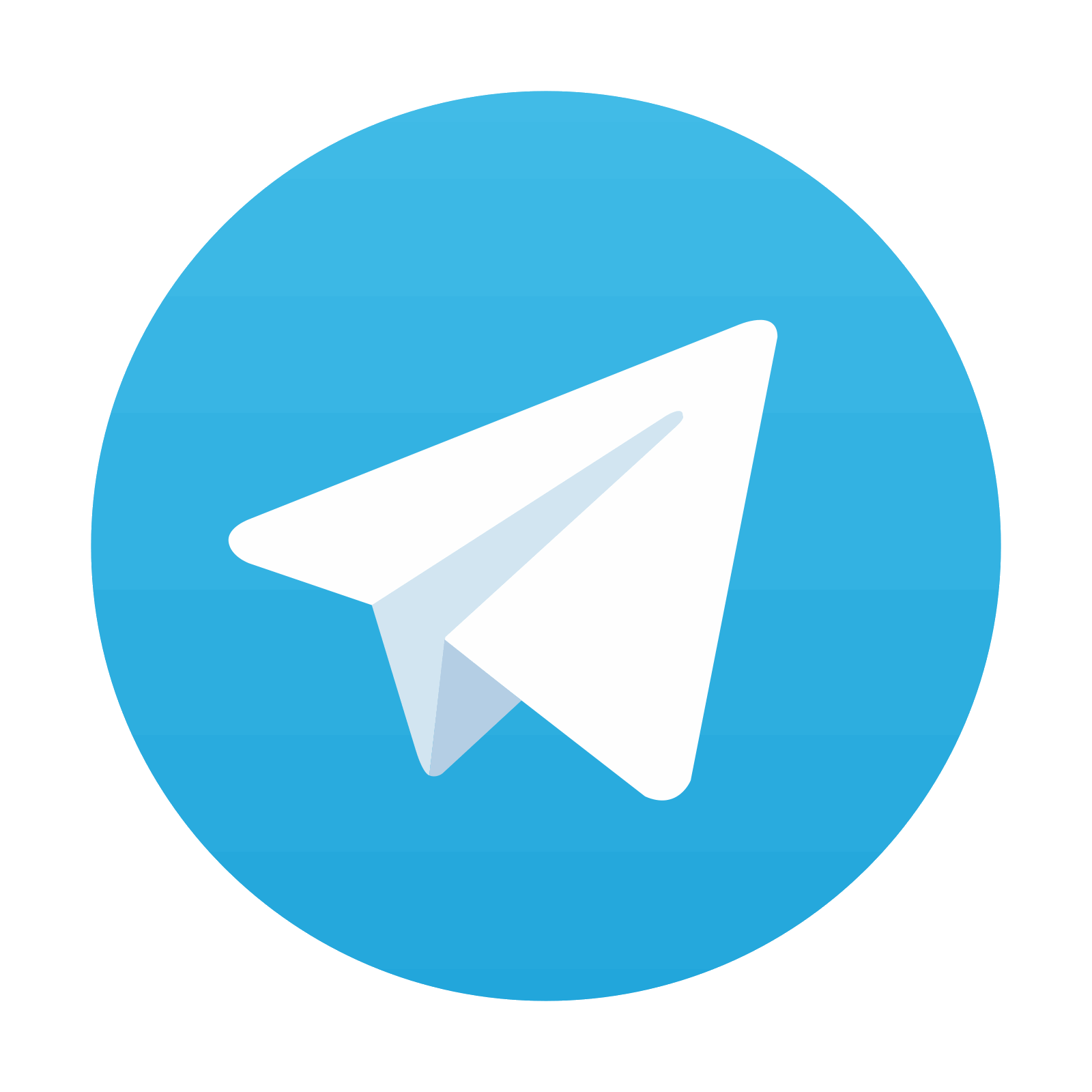
Stay updated, free articles. Join our Telegram channel
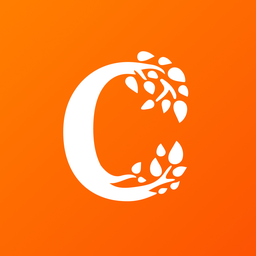
Full access? Get Clinical Tree
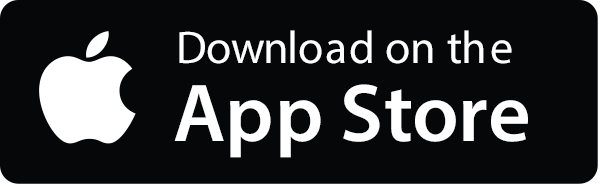
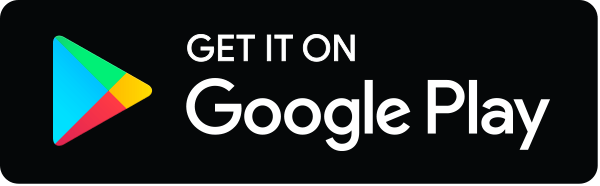