Luc L. Mertens and Mark K. Friedberg The Hospital for Sick Children; University of Toronto, Toronto, ON, Canada Evaluation of systolic function is an essential part of every echocardiographic examination. This should include evaluation of global as well as regional myocardial function. Various echocardiographic techniques have been used for quantification of left ventricular function and have been well established in adult cardiology. For children and patients with congenital heart disease, both the acquisition and the interpretation of the various measurements can be more challenging related to variability in ventricular shape, size, and loading conditions. In this chapter we focus on echocardiographic techniques that can be used for the assessment of left ventricular systolic function. Cardiovascular function is determined by the interaction between the heart and the systemic and pulmonary circulations. The cardiovascular system should generate sufficient cardiac output to provide a level of tissue oxygenation that is commensurate with the tissue oxygen demand. The heart is the driving force of the circulatory system and can be considered an adaptive volume pump that, within certain limits, can adjust to changes in loading conditions defined as the need to pump higher volumes (volume loading) or overcome higher resistances (pressure loading). Cardiac pump function is dependent on active myocardial force development resulting in pressure build‐up in the ventricular cavity early in systole. This rapid pressure increase occurs very early on in systole while both the atrioventricular and semilunar valves are closed (isovolumic contraction). Once the pressure in the ventricle exceeds the pressure in the great artery, the semilunar valve opens, and myocardial fiber shortening and wall deformation lead to ejection of blood. The relationship between force or pressure development, at the one hand, and myocardial deformation and ejection, on the other hand, can be described at different physiological levels as illustrated in Figure 7.1 [1]. Myocytes are organized in fibers, and at this level sarcomere activation through electromechanical coupling results in active myocardial force development and fiber shortening. The myocardial wall is composed of fibers organized in different directions, and at this level segmental force development increases regional wall stress and causes myocardial shortening in longitudinal and circumferential directions, depending on the fiber orientation. The shortening of fibers oriented in different directions results in wall thickening in the radial direction. At the ventricular level, the increase in ventricular cavity pressure and – after opening of the semilunar valve – the shortening of the myocardial segments results in the displacement and ejection of blood out of the cavity. The relationship between cavity pressure changes and volume changes has been studied invasively by pressure–volume loops. The end‐systolic pressure–volume relationship, or elastance, is considered the gold standard for evaluating contractile function, as this parameter is independent of loading conditions, thereby representing intrinsic myocardial contractility. The complex structural organization of myofibers in different layers is one of the basic determinants of cardiac mechanics. In the normal heart, sarcomeric shortening is approximately 15%. This relatively small shortening percentage results in a 35–40% wall thickening and ejection of 55–60% of ventricular blood volume in each systole [2,3]. In contrast to skeletal muscle fibers, cardiac fibers do not assemble in parallel arrays but bifurcate and recombine to form a complex 3D network. In the left ventricular subepicardium, myofibers are organized in a left‐handed obliquely oriented spiral, changing to a more circumferential orientation in the LV mid‐wall and to a right‐handed spiral of more obliquely and longitudinally oriented fibers in the subendocardial layer. This change in fiber orientation is progressive throughout the LV wall but results in the epicardial and endocardial fibers being oriented more longitudinally while the middle LV layers are oriented in a more circumferential orientation. Myocardial function is most commonly described in a spatial Cartesian reference frame with three major axes describing the direction of muscle shortening: longitudinal, circumferential, and radial (Figure 7.2). The complex 3D myocardial fiber organization is a major component increasing mechanical efficiency as fiber interaction and shear forces during systole result in cross‐fiber shortening, which is responsible for the amplification of the limited 15% myofiber shortening. In the LV, the mid‐wall layer is the most prominent, explaining the predominantly circumferential shortening. There are no fibers running in the radial direction. Radial thickening can be explained by the thickening of the fibers running in circumferential and longitudinal directions. As the fibers shorten in both directions, this causes wall thickening in the radial direction. The more longitudinally oriented subepicardial and subendocardial fibers cause longitudinal shortening with displacement of the atrioventricular annular plane in the direction of a relatively stationary cardiac apex. This longitudinal annular motion can be described and studied as a parameter of longitudinal function. Whereas in the LV circumferential shortening predominates during ejection, in the RV longitudinal shortening is the dominant myocardial motion during ejection. In the LV, the opposite spiraling of the oblique subendocardial and subepicardial fibers causes a torsional motion of the heart caused by clockwise rotation at the base (Video 7.1) and counterclockwise rotation of the apex when viewed from the apex (Video 7.2). This wringing motion of the heart contributes to cardiac output and is an important component of cardiac mechanics. The untwisting occurring during early diastole has been demonstrated to be an important determinant of early cardiac filling and diastolic function. For a more detailed overview on the basics of cardiac mechanics as related to echocardiography, we refer to two more comprehensive reviews [4,5]. An understanding of cardiac mechanics is essential for interpreting myocardial deformation imaging based on speckle‐tracking echocardiography (STE) technology [6]. Figure 7.1 Cardiac function can be studied at different levels (myocyte, myocardial wall segments, or the ventricle as a whole). At each level, force development results in myocardial deformation, which at the organ level is responsible for generating cardiac output. EES, elastance at end‐systole; EF, ejection fraction; FS, fractional shortening. Figure 7.2 Myocardial fiber orientation and myocardial deformation. By convention myocardial motion is described using a Cartesian coordinate system. More longitudinally oriented fibers cause longitudinal shortening while more circumferentially oriented fibers cause circumferential shortening. The combined circumferential and longitudinal shortening results in radial wall thickening. Spiraling of the left ventricular fibers is responsible for the rotational motion with clockwise rotation of the base and counterclockwise motion of the apex when viewed from the apex. Global ventricular performance is the ability of the heart to develop force and deform so that it can eject blood. Ventricular performance can be assessed by measuring the stroke volume, which is the amount of blood volume ejected by the heart with each heartbeat. Cardiac output is the product of stroke volume and heart rate and varies with changes in preload, afterload, heart rate, and intrinsic contractility. Preload is the load present at end‐diastole before the onset of cardiac contraction. In an isolated muscle strip model it is defined as end‐diastolic fiber length. Increased end‐diastolic fiber length results in increased force development or contractility, up to a maximal length above which there is a decrease in force development. This adaptive mechanism is very useful in adjusting to physiologic fluctuations of venous return. An acute increase in venous return normally leads to fiber lengthening at end‐diastole, and the increased contractile function will result in increasing stroke volume. Reduced preload lowers stroke volume and reduces ejection. The relationship between preload and contractility is called the Frank–Starling relationship. In the intact organ, end‐diastolic fiber length cannot be measured, and LV end‐diastolic volume or pressure is usually used as a surrogate marker for fiber length or LV preload. In patients with chronic LV volume loading (e.g., those with a ventricular septal defect (VSD) or patent ductus arteriosus (PDA)), ventricular remodeling with eccentric hypertrophy occurs. This results in end‐diastolic volume becoming an inaccurate measurement of preload as the increased resting length of the myofibers reduces end‐diastolic fiber stress. In patients with a significant left‐to‐right shunt, eccentric remodeling can thus normalize preload and LV volume measurements should not be used as a substitute for preload. Likewise, end‐diastolic pressure does not accurately reflect preload, as it is influenced by ventricular compliance and thus does not directly reflect fiber length. In a stiffer ventricle, higher end‐diastolic pressure does not reflect higher preload. Preload assessment thus is challenging in the intact organ, especially in congenital heart disease where chronic volume loading can result in eccentric ventricular remodeling. Fiber length cannot directly be assessed by ultrasound, and there is no good surrogate parameter directly reflecting preload status. This makes assessment of systolic function challenging in the presence of changing preload conditions, which occurs very often in congenital heart disease. The presence of left‐to‐right shunts at a post‐tricuspid level can result in volume loading of the LV. Also, significant mitral and aortic regurgitation are associated with LV volume loading. Chronic volume loading in the presence of preserved contractile function typically results in an increase in functional parameters (such as shortening and ejection fractions), while acute volume unloading, such as after VSD or PDA closure, results in a sudden decrease in these same LV functional parameters. In an isolated muscle strip experiment, afterload is defined as the force opposing muscle shortening after the onset of contraction. Increased afterload reduces the total amount of myocardial shortening as well as the velocity of myocardial shortening. Thus, afterload must be taken into account when interpreting systolic functional indices during the ejection phase, as both the extent of myocardial shortening and the velocity of shortening are expected to be influenced by afterload changes. Important determinants of afterload are peak arterial pressure, arterial compliance or vessel stiffness, and vascular resistance. In the intact organ, afterload is best defined by wall stress [7], which is determined by intracavitary pressure, cavity dimension, and wall thickness according to Laplace’s law (Figure 7.3): Figure 7.3 Definition of wall stress by the Laplace formula. Global left ventricular (LV) wall stress increases with intracavitary pressure and with an increase of left ventricular end‐diastolic dimension (LVEDD), and with a decrease of wall thickness. Regional wall stress is influenced by the curvature of the wall defined by the radius of curvature. This is the radius of the circle that fits the local wall curvature. At the LV apex the radii of curvature are smaller, resulting in lower local wall stress, while at the base the wall is flatter with higher values for radii of curvature. The base of the interventricular septum has the largest radius of curvature, resulting in higher wall stress. Wall stress varies throughout systole. Peak systolic wall stress is the maximal wall stress occurring during systole. When chronically elevated, such as in arterial hypertension, this is the trigger for the development of concentric hypertrophy. Total systolic wall stress is reflected by the time–stress integral, which determines myocardial oxygen consumption. End‐systolic wall stress is the afterload at the end of systole at the time of aortic valve closure and influences the amount of myocardial systolic shortening. Wall stress is a force that can be represented as a vector with components in different directions. Again, the Cartesian cardiac coordinate system can be used as the reference, with circumferential stress (short axis), meridional stress (long axis), and radial stress (transmural) as the main stress vector components. Due to the structural organization of the myofibers, fiber stress is not identical to stresses measured in the cardiac coordinate system as fibers are not perfectly aligned with the Cartesian system [7]. As fiber orientation is difficult to measure, measuring fiber stress as a measure of afterload is practically impossible. In clinical practice, ventricular systolic pressure or blood pressure is often used as a surrogate measure for afterload. This is a gross simplification as ventricular pressure is only one of the parameters influencing wall stress. An acute increase in systolic pressure will, however, result in an immediate increase in wall stress and thus afterload. A chronic increase in afterload causes concentric ventricular remodeling with addition of myofibers in the circumferential direction resulting in wall thickening. A thicker wall reduces wall stress and thus afterload [8]. This implies that in a remodeled ventricle with increased wall thickness, pressure does not directly reflect afterload. When compensated hypertrophy is present, afterload may be normal despite increased pressure. Therefore, ventricular pressure should be used cautiously as a surrogate for afterload. Also, cavity size influences wall stress through changes in wall curvature as reflected by the radii of curvature. A flatter wall has a larger radius of curvature compared to a more curved one and results in higher wall stress. This explains why ventricular dilation in dilated cardiomyopathy increases afterload as the loss of ventricular curvature increases the radii of curvature and thus local wall stress [9,10]. Thus, LV dilation in response to decreased contractility results in increasing cardiac output (related to higher ventricular volume) at the expense of increased afterload. Beyond preload and afterload, heart rate is an important determinant of cardiac performance. There is a curvilinear relationship between heart rate and contractility with an increased heart rate resulting in increased myocardial force development. This physiologic relationship is termed the “force–frequency relationship,” and is related to increased efficiency of intracellular calcium metabolism at higher heart rates [11]. However, when the heart rate becomes too high, the maximal capacity of the calcium cycling mechanisms is exceeded, which results in a decrease of contractile force above a maximal heart rate (typically >180 beats/min for the adult heart). In isolated muscle strips obtained from failing ventricles, the force–frequency response is typically blunted, thus an increase in heart rate is no longer increasing contractile function and the responsive sinus tachycardia may be detrimental [12]. Additionally, the faster heart rate decreases ventricular filling duration and, especially in the presence of diastolic dysfunction, the decreased filling can further reduce cardiac output. When interpreting cardiac function by echocardiography, the impact of heart rate on contractility should be taken into account, especially in children where heart rates can vary significantly between different studies. Intrinsic contractility refers to the ability of the cardiac muscle to actively develop force and to shorten independent of preload, afterload, and heart rate. In the isolated myocardial fiber, increased contractility results in a higher myocardial shortening velocity and a higher percentage of total shortening. Intrinsic contractile function would be an excellent parameter for assessing myocardial health but is extremely difficult to assess in the intact organ. As contractility is influenced by loading conditions and heart rate, it remains elusive to study independently from both. Invasive recording of pressure–volume loops combined with preload manipulation is the gold standard for contractility assessment but is a complicated invasive technique that has never been clinically implemented [13,14]. For interpretation of systolic function it is essential to realize that loading conditions influence all echocardiographic parameters, which is a limitation if you want to assess myocardial contractility. From a clinical perspective it is, however, more important to study ventricular performance as this represents how the heart is responding to variable loading conditions. Measurements of ventricular performance can be used in clinical follow‐up and longitudinal assessment, taking into account changes in loading conditions and heart rate in the interpretation of the measurements. For instance, a decrease in left ventricular ejection fraction is often observed in infants undergoing VSD or PDA closure. This does not reflect an acute deterioration in contractile function but is related to an acute decrease in preload related to removal of the left‐to‐right shunt and increased afterload, as the LV is no longer exposed to the low‐resistance pulmonary circulation. Apart from these traditional parameters other physiologic factors influence ventricular performance. These include synchronicity of contraction and ventriculo‐ventricular interactions. Both can significantly impact global function also in children. Normal global left ventricular function requires a coordinated contraction between different segments that is determined by the electrical activation wave that exits the conduction tissue at the septal apical part, with fast propagation of the electrical depolarization wave from the apical part to the basal lateral wall. Mechanical dyssynchrony can result from electrical conduction delays, such as left bundle branch block, or from regional myocardial dysfunction. Left bundle branch block typically results in early septal activation with delayed activation of the lateral wall. Early septal activation pre‐stretches the LV lateral wall which increases the force developed once activated. This imbalance in force development stretches the septal shortening, resulting in a typical septal motion with early shortening followed by stretching (septal flash). Mechanical dyssynchrony causes overall contractile inefficiency and is a mechanism that contributes to progressive ventricular dysfunction and can result in heart failure. It can be studied by measuring the timing of systolic mechanical activation in different myocardial segments by various techniques including color tissue Doppler and myocardial deformation imaging [15]. In appropriately selected cases, cardiac resynchronization therapy can result in improvement of cardiac function also in children [16]. Studying electromechanical dyssynchrony in patients with conduction abnormalities and dysfunction can help to identify a possible treatable cause of ventricular dysfunction and heart failure and should always be considered. Another important determinant of ventricular function in patients with congenital heart disease is the influence of ventriculo‐ventricular interactions on regional and global ventricular function [17]. In the normal circulation the two ventricles interact as they are functioning in series, and the output of one ventricle will influence filling of the other. The interventricular septum, the pericardium, and shared fibers between both ventricles further contribute to ventricular interactions. These interactions can be important to consider in the assessment of ventricular function in specific conditions. For instance, in patients after tetralogy of Fallot repair with severe pulmonary regurgitation, the dilated right ventricle (RV) together with the presence of right bundle branch block and dyssynchronous RV activation can influence LV filling and consequently LV output. In children, maturational myocardial changes should also be considered. In the newborn period, particularly in preterm infants, the myocardial contractile apparatus and calcium metabolism are immature, resulting in decreased systolic and diastolic functional reserve. Cardiac output increase in infants is more dependent on heart rate as ventricular volumes are small. During growth, the myocardium matures and progressively thickens, especially during adolescence. This influences cardiac functional parameters over time and, for every parameter used, the physiologic influence of cardiac growth and development must be studied. A wide variety of echocardiographic measurements have been proposed as indices of LV function. The first group of measurements measures changes in LV chamber size and wall thickness that occur during systole. These include one‐dimensional calculation of LV fractional shortening (FS, also known as shortening fraction) and 2D or 3D calculation of LV ejection fraction (EF). Wall thickening can be studied by M‐mode or by studying radial thickening by myocardial deformation imaging. A second group of measurements are based on Doppler measurements and include timing measurements such as peak dP/dt, the myocardial performance index, as well as tissue velocity measurements obtained by tissue Doppler. A third group of measurements aim at quantifying myocardial deformation in the principal directions of contraction with an emphasis on assessment of LV longitudinal shortening based on speckle‐tracking algorithms. A distinction between global and regional measures of LV function has to be made. LV EF, dP/dt, and global longitudinal strain (GLS) measurements quantify global LV function. Tissue Doppler velocities and segmental LV strain measurements quantify regional myocardial function. Traditionally, assessment of regional myocardial function was based on subjective scoring of regional wall motion or thickening. Techniques such as measurement of tissue velocities in different myocardial segments and quantification of regional myocardial deformation by strain imaging allow quantification of regional myocardial function. Regional wall motion abnormalities are very common in ischemic heart disease but can also be detected in patients with cardiomyopathies and valvar disease, and in congenital heart disease. Two different phases need to be identified during systole. In the normal heart, force development starts prior to ejection and peaks in the first one‐third of systole. The time between electrical activation and the opening of the semilunar valve is called the isovolumetric contraction period. In the absence of mitral regurgitation this phase is isovolumetric and as the aortic valve is still closed, the pressure development is not influenced by afterload. Measurements made in this period are considered to be less load dependent. These include measurements such as pre‐ejection time duration or isovolumetric acceleration based on tissue Doppler or strain rate. These measurements tend to be more variable as the isovolumetric period is very short, requiring high temporal resolution, particularly at high heart rates. This explains why most echocardiographic parameters are measured during the ejection phase, which makes the measurements intrinsically afterload dependent. When using functional parameters in clinical practice the feasibility, reliability, and accuracy of the measurements should be considered. The feasibility of a measurement reflects whether a measurement is technically possible. For example, due to limitations in echocardiographic windows, the feasibility of a 3D volumetric acquisition is generally lower than a 2D acquisition, especially in obese or postoperative patients with poor ultrasound windows. The reliability of a measurement is represented by its reproducibility. When the same measurement is repeated by the same observer, this is the intraobserver variability, which is generally lower than the interobserver variability (i.e., the variability between different observers). Knowing the variability of a measurement is crucial to be able to interpret when a measurement difference can be considered as reflecting a true change in function (minimal clinically relevant difference). For example, if a 10% decrease in ejection fraction is measured by the biplane Simpson method, the measurement variability must be known in order to determine whether this difference is related to measurement variability or whether it reflects a true change in function. Measurement variability can be reduced by standardization and automation of acquisition and analysis techniques. Published quantification guidelines can be used as an important tool for standardizing scanning protocols and measurements [18,19]. Each echocardiographic laboratory should ideally define standard scanning protocols, optimize measurements, and evaluate their reliability. The accuracy of a measurement reflects whether the measurement indicates what it is supposed to measure. The accuracy of a measurement can be determined by validating the technique against a gold‐standard method. For example, LV echocardiographic 3D volumetric measurements have been validated against volumetric measurements derived from cardiac magnetic resonance imaging (MRI), with MRI measurements considered to be gold‐standard method against which the echocardiographic measurements are to be compared. Fiber shortening during ejection results in displacement of blood and dimensional changes both within the cavity and within the myocardial walls. Traditionally, the assessment of global systolic function has been based on measuring changes in ventricular size and volume during the systolic ejection phase from apical, long‐axis, and short‐axis views. Measurement of dimensional changes can be based on one‐dimensional (M‐mode), 2D (area‐length, biplane Simpson method), or 3D measurements (Table 7.1). The American Society of Echocardiography published separate guideline papers on the quantification of chamber sizes and dimensions in the adult [18] and pediatric age groups [19]. Left ventricular dysfunction is commonly associated with LV remodeling, and measurement of LV dimensions should be a routine part of systolic functional assessment. Typically, progressive LV dysfunction causes LV dilation, which is a mechanism for maintaining stroke volume, as a larger ventricle needs to contract less to pump the same blood volume. LV dilation causes a more spherical LV wall configuration, which results in increased wall stress or afterload, especially in the apical segments. Therefore, diagnosis of LV dysfunction requires investigation of LV dimensions and remodeling. Typically, this involves measuring the LV end‐diastolic dimension (LVEDD z‐score) and the LV septal and posterior wall thickness (LVPWT z‐score) from a parasternal short‐axis view using M‐mode or 2D measurements (Figure 7.4). The LV length can be measured from an apical four‐chamber or subxiphoid long‐axis view. Derived parameters include the sphericity index and the thickness‐to‐dimension ratio. The sphericity index can be defined as the LV minor or short‐axis dimension divided by the LV major‐axis dimension or LV length. At end‐diastole this parameter is normally 0.6–0.7 but approaches 1 as the ventricle becomes more spherical as typically occurs in dilated cardiomyopathy [20]. In patients after anthracycline exposure the thickness‐to‐dimension ratio has been described to change before changes occur in myocardial performance, thereby reflecting ventricular remodeling [21]. Progressive LV dilation, wall thinning, and a more spherical configuration represent unfavorable ventricular remodeling, while the opposite is seen in reverse remodeling. These parameters are very useful in defining disease progression. The most commonly used parameters for assessing left ventricular function are based on changes in chamber size from end‐diastole to end‐systole. End‐diastole is best defined as the image frame before mitral valve closure and end‐systole as the frame before mitral valve opening. M‐mode echocardiography was one of the first techniques available for assessing LV dimension changes. Either a long‐axis or short‐axis cut through the LV just below the mitral valve leaflet tips is obtained. A short‐axis cut is our method of choice as it is easier to evaluate whether the imaging plane is truly perpendicular to the LV long axis, with the LV appearing as a circular structure in systole and the RV projecting anteriorly to the LV (Figure 7.4). This requires an optimal parasternal position of the transducer with a scanning plane perpendicular to the plane of interest. M‐mode echocardiography has the advantage of having a high temporal resolution compared to 2D imaging. The spatial resolution of 2D echocardiography, however, is better. Two‐dimensional imaging allows for direct assessment of the segments being measured, and it also has the ability to evaluate for in‐plane motion artifact. Therefore, the American Society of Echocardiography pediatric quantification guideline document recommended using a 2D short‐axis view at the level of the papillary muscles for the measurement of LV cavity dimensions and calculation of fractional shortening [19]. The disadvantage of using 2D images is the lower temporal resolution, which is a limitation in infants at higher heart rates. The 2D method also has higher measurement variability compared to M‐mode measurements. Shortening fraction (SF) is defined as the percent change in left ventricular dimension from end‐diastole to end‐systole based on either M‐mode or 2D imaging acquired just below the level of the mitral valve leaflet tips. SF represents the degree of apposition of the basal LV posterior wall to the basal ventricular septum. As the mitral valve cannot be viewed on the short‐axis cut, the left ventricular cavity is measured at the end of diastole, defined as the onset of the QRS‐complex (LVEDD), and at end‐systole, which is the closest point of apposition between the septum and the posterior wall (left ventricular end‐systolic dimension, LVESD). Shortening fraction is expressed as a percent and is calculated as: Table 7.1 Left ventricular systolic function derived from dimensional changes 2DE, two‐dimensional echocardiography; 3DE, three‐dimensional echocardiography; MAPSE, mitral annular plane systolic excursion; Vcfc, velocity of circumferential fiber shortening corrected for heart rate. Figure 7.4 M‐mode calculation of left ventricular (LV) shortening fraction. A short‐axis M‐mode is obtained at the basal level of the ventricle just distal to the mitral valve leaflet tips. LV end‐diastolic and end‐systolic dimensions are measured and shortening fraction can be calculated. Shortening fraction normally varies between 28% and 38%. Values below 28% suggest reduced systolic LV function, while values above 38% indicate hyperdynamic LV function. The popularity of this measurement (in particular the M‐mode measurement) is largely due to the ease with which it can be obtained. There are, however, important limitations to its use. One limitation is that SF reflects thickening of the basal part of the posterior wall and ventricular septum and thus by definition is a regional basal parameter which is used to assess global LV function. SF only reflects global pump function in the absence of regional wall motion abnormalities. Also, motion in the basal ventricular septum can be influenced by different factors. A typical example is septal dyskinesia in the presence of right ventricular volume loading. This can be associated with a paradoxical systolic motion of the basal ventricular septum moving away from the posterior wall. In this instance SF cannot be measured. Similarly, immediately after cardiopulmonary bypass, septal hypokinesia or dyskinesia is a common finding, and SF often underestimates global LV systolic function in the immediate postoperative period. When regional myocardial function is preserved in the septum and posterior walls but reduced in other segments, SF overestimates global LV performance. Likewise, SF mainly reflects circumferential fiber shortening and, as such, does not fully account for longitudinal shortening. Loading conditions should also be considered when interpreting SF measurements. As an ejection phase parameter, SF is influenced by changes in LV volume and pressure loading. Volume loading will generally result in increased SF. In the presence of chronic mitral or aortic regurgitation, a normal – and certainly a reduced – SF should be considered as a possible sign of underlying LV dysfunction. Increased afterload results in a decrease in SF. A typical example is a hypertensive crisis, which causes an acute increase in arterial pressure and LV afterload, which typically results in reduction in SF. This is not due to intrinsic myocardial dysfunction but is related to sudden changes in loading conditions. Blood pressure control will typically result in normalization of systolic pump performance. An additional limitation is the dependency of SF measurements on LV geometry. Typically, LV hypertrophy without LV dilation, as occurs in hypertrophic cardiomyopathy, results in a smaller LV cavity. In this context, changes in LV dimensions during systole result in overestimation of LV systolic function. Data are available on the reliability of SF measurements. In a multicenter study, Lipshultz et al. compared M‐mode measurements obtained in local laboratories with core lab measurements [22]. They found the highest interclass correlations (ICC) for LVEDD (ICC 0.97) but a lower value for SF (ICC = 0.64). This is not surprising as SF measurements are based on measurement of two parameters (LVEDD and LVESD), increasing measurement variability. A recent study from the Pediatric Heart Network z‐score group demonstrated that 2D‐based assessment of SF can be highly variable in normal children [23]. Data also showed that in children with dilated cardiomyopathy measurements of dimensions by 2D were generally more variable compared with measurements of ventricular areas [24]. A second parameter based on measurement of dimension changes is the measurement of LV ejection fraction (EF), which is defined as the percentage change in LV volume from end‐diastole to end‐systole. Its calculation requires measurement of LV volumes: where LVEDV is the LV volume at end‐diastole and LVESV the LV volume at end‐systole. LV volumes can be calculated by various methods. The first is based on measurement of LV dimensions using M‐mode echocardiography. Quinones et al. [25] proposed a simplified formula to calculate EF by measuring internal LV dimensions and based on simplified geometrical assumptions of LV shape. This method has all the limitations of M‐mode echocardiography outlined in the previous paragraph, the most important being that it measures changes in only two segments. The pediatric quantification guidelines recommend two different methods based on 2D echocardiography. The method most commonly used and also recommended by the American Society of Echocardiography adult guidelines is the modified Simpson or disk summation method [26,27]. In this method two nearly orthogonal planes from the apical view are used (apical four‐ and two‐chamber views). The LV is divided into a number (n) of cylinders or disks of equal height. The height of each cylinder is determined by dividing the total length of the LV cavity (L) by the number of cylinders (L/n). The volume of each cylinder is calculated from the diameter of the cylinders (ai and bi) obtained in the two planes. The LV volume is the sum of the volumes of the individual cylinders using the formula: Figure 7.5 Biplane modified Simpson calculation of left ventricular ejection fraction measured in a child with dilated cardiomyopathy. In the apical four‐ and two‐chamber views, the endocardial borders are manually traced; end‐diastolic volume, end‐systolic volume, and ejection fraction are calculated using the modified Simpson algorithm. Figure 7.6 Area–length method for calculation of left ventricular (LV) ejection fraction (EF). In this method LV basal areas are measured from the subxiphoid or parasternal basal short‐axis views, while LV length can be measured from the apical four‐chamber or subxiphoid long‐axis views. The measurements are obtained in end‐systole and end‐diastole, and EF is calculated using the area–length formula. The summation of disks method is probably the most reliable for calculating EF, but also the most time‐consuming in clinical practice because it requires LV endocardial border tracing at end‐diastole and end‐systole in two apical views (Figure 7.5). In patients with poor echocardiographic windows and also in severely dilated ventricles, endocardial border detection and tracing can be challenging. The LV lateral and anterior walls can be especially difficult to trace. This influences both the feasibility and the reliability of this method. Another simplified method for calculating LV volumes is the “bullet,” or area–length, method. This method is possibly more reproducible than the biplane Simpson method, but it is influenced by changes in LV shape. The area–length method requires measuring the short‐axis basal LV area and the LV length. The LV basal area can be obtained from a parasternal or subxiphoid short‐axis view (Figure 7.6). LV length can be measured from the apical four‐chamber or the subxiphoid long‐axis views. LV volume can then be calculated using the formula: The measurements need to be repeated in end‐diastole and end‐systole. The measurement of LV length can be challenging and variable between observers. The LV apex is trabeculated, especially in newborns, and this influences the apical measurement. The preferred 2D method for measurement of LV EF is currently uncertain and largely dependent on individual laboratory experience and practice. For both methods optimal 2D images of the LV are required and foreshortening of the LV cavity should be avoided. Generally, the variability of the measurements is within the 10–15% range. A reduction in EF of >10% in the normal range (>55%) and >5% if EF <55%, is considered clinically significant. Automated border detection algorithms, including those using artificial intelligence, have been developed to reduce measurement variability arising from manual border tracing, and these more automated techniques have been integrated in ultrasound systems [28,29]. The more recent versions of the automated methods are based on speckle‐tracking technology (Figure 7.7). In adults, these automated methods significantly reduce interobserver variability and post‐processing times, but the methods have not been well validated in children [30]. Three‐dimensional echocardiography has recently been introduced, and the availability of high‐frequency matrix transducers allows the acquisition of high‐resolution full volumetric datasets also in children [31]. Most 3D analysis techniques for EF are currently largely automated, thereby reducing measurement variability and increasing accuracy (Figure 7.8, Video 7.3). LV 3D volumes have been demonstrated to be more accurate than 2D when compared with LV volumes measured by MRI [32]. The advantage of 2D and 3D EF calculations is that they account for regional wall motion abnormalities and are the methods of choice so long as image quality is adequate. It should be remembered that whatever method is used, EF is load dependent, and 2D based methods especially are dependent on ventricular geometry [33–36]. Figure 7.7 Automated methods for left ventricular (LV) ejection fraction (EF) calculations. Instead of manual tracing of the borders in the apical two‐ and four‐chamber views, an automated border detection method is used to calculate LV volumes and ejection fraction. EDV, end‐diastolic volume; ESV, end‐systolic volume. A separate assessment of longitudinal fiber shortening provides important additional information on LV function. In the normal LV, the circumferentially oriented fibers predominate over the longitudinal fibers and short‐axis shortening exceeds long‐axis shortening. Therefore, LV sphericity normally decreases during contraction as the LV short axis decreases more than its long axis. Long‐axis function is, however, an important contributor to LV ejection and global function. As subendocardial fibers contribute to longitudinal contraction, subendocardial dysfunction or fibrosis will affect longitudinal LV function more than circumferential contraction. Pressure loading also affects longitudinal function more than circumferential function. Both factors probably explain why patients with aortic valve stenosis have more significant abnormalities in longitudinal than radial function [37]. Different techniques can be used to quantify longitudinal LV function. The easiest measurement is the displacement of the mitral valve annulus, also called mitral annular plane systolic excursion (MAPSE). MAPSE can be obtained from the apical four‐ and two‐chamber views by M‐mode echocardiography (Figure 7.9). An M‐mode cursor can be placed through the lateral, septal, anterior, and/or posterior walls [38], although generally either the septum or the lateral wall is preferred. The systolic excursion is measured from the “lowest” point in diastole to aortic valve closure. In adults, MAPSE ranges between 10 and 15 mm, and a value <8 mm is considered abnormal. MAPSE is heart‐size dependent, and unfortunately pediatric normal values are not available, limiting its use in younger children. In patients with increased LV afterload, including arterial hypertension and aortic stenosis, reduced longitudinal function can be present despite a preserved EF, suggesting that reduced longitudinal function may be an early sign of ventricular dysfunction. Diseases associated with increased mass‐to‐volume ratio (such as arterial hypertension, aortic stenosis, or hypertrophic cardiomyopathy) are associated with increased short‐axis shortening and decreased long‐axis function. MAPSE is load dependent, and it is influenced by valve regurgitation and by translational motion as the heart moves in the chest during contraction. Newer methods such as tissue Doppler and longitudinal strain measurements also provide measurements of LV longitudinal function and are discussed later in this chapter. For a complete LV functional assessment, at least one of these techniques to assess longitudinal LV function should be performed. Figure 7.8 Three‐dimensional echocardiography for left ventricular (LV) ejection fraction calculation. A full 3D dataset is acquired and an automated analysis of LV volumes and ejection fraction can be performed. Figure 7.9 Mitral annular plane systolic excursion (MAPSE). An M‐mode cursor is placed through the mitral annulus aligned with the longitudinal motion of the annulus during systole. The excursion of the annulus during systole is measured. We use color tissue Doppler to help with the timing of the measurements. To overcome some of the problems associated with load‐dependency of SF and EF, methods correcting dimensional changes for afterload have been developed. One of these measures the velocity of circumferential fiber shortening (Vcf), i.e., the velocity of LV dimension change during ejection calculated by dividing the FS by the LV ejection time (Vcf = FS/ejection time). Vcf is an estimate of the average rate of change of the LV diameter per unit of time. Typically, ejection time is calculated from an M‐mode through the aortic valve, thereby utilizing the high M‐mode temporal resolution (around 1500 frames/s). This measurement reflects the velocity at which shortening occurs. As a timing parameter it has to be corrected for heart rate, which can be done by dividing the SF measurement by the square root of the preceding R‐R interval (Bazett’s correction):
CHAPTER 7
Left Ventricular Systolic Function Assessment
Introduction
Mechanics of cardiac systolic function
Fiber organization and wall mechanics
Cardiac function at the ventricular level
Echocardiographic methods of left ventricular systolic function assessment
Systolic function derived from dimensional changes
Left ventricular dimensions and shape representing left ventricular remodeling
Dimensional changes in systole representing left ventricular performance
Dimensional changes
Strengths
Weaknesses
Shortening fraction by M‐mode
Ease of measurement
Only reflects basal regional function
Influenced by abnormal septal motion
Does not measure longitudinal shortening
Load dependent
Geometry dependent
Shortening fraction by 2DE
Less dependent on probe positioning on chest and angulation than M‐mode
Reflects basal regional function
Influenced by abnormal septal motion
Does not measure longitudinal shortening
Load dependent
Geometry dependent
Ejection fraction by biplane Simpson
Is a global parameter as it takes regional function into account
Endocardial border detection can be challenging
Load dependent
Ejection fraction by 5/6 area × length
Endocardial border detection in short axis easier than biplane Simpson method
Geometry dependent
Load dependent
Ejection fraction by 3DE
More accurate than 2DE EF methods
Load dependent
MAPSE by M‐mode
Ease of measurement
Measures longitudinal function
Load dependent
Influenced by valve regurgitation
Vcfc
Relatively preload independent
Based on geometric assumptions
Afterload dependent
Longitudinal left ventricular systolic function
Correcting dimensional changes for afterload
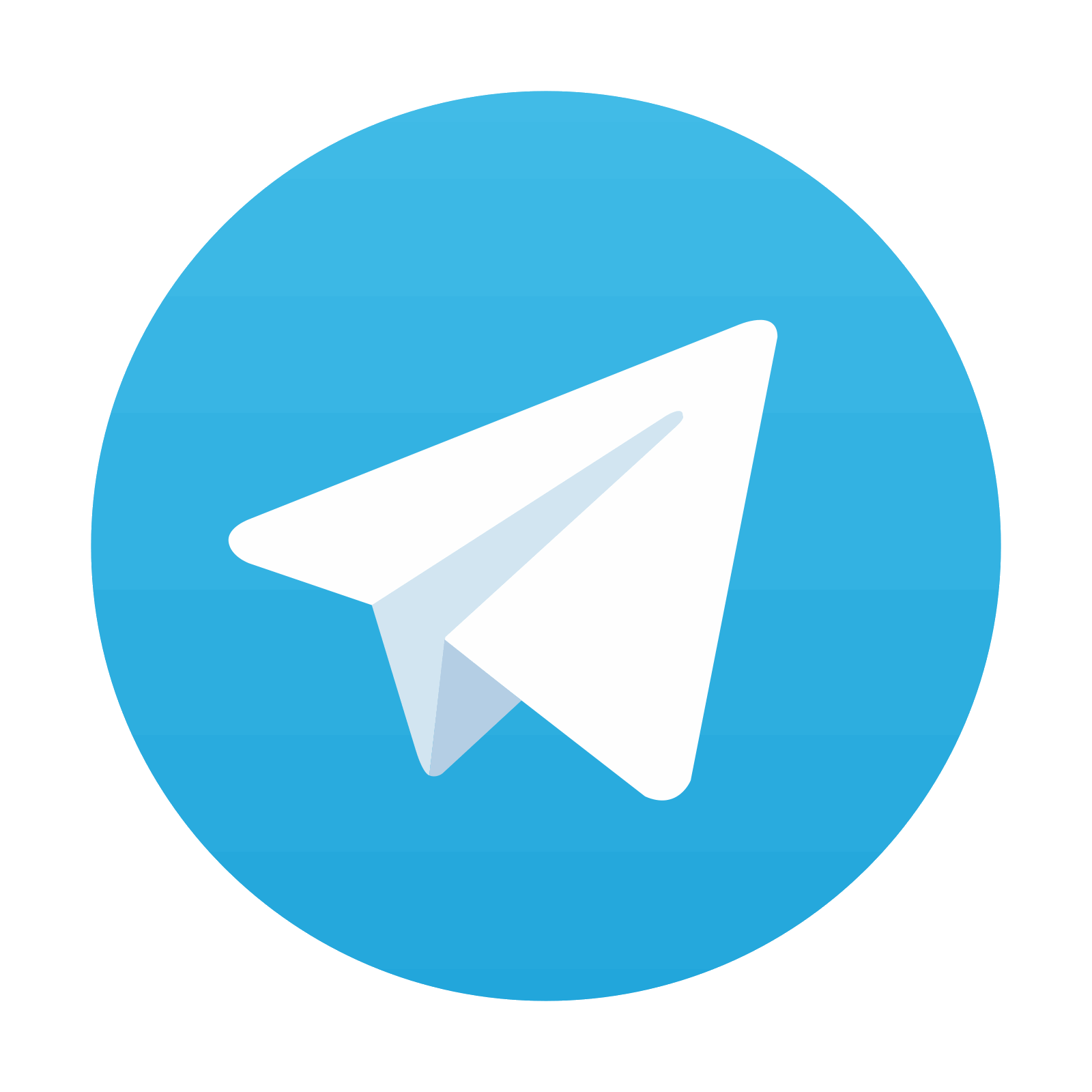
Stay updated, free articles. Join our Telegram channel
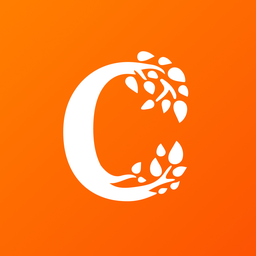
Full access? Get Clinical Tree
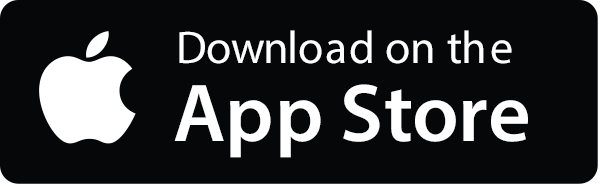
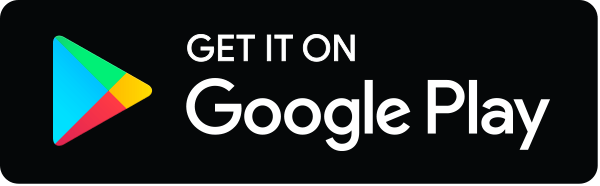