Pei‐Ni Jone Children’s Hospital Colorado; University of Colorado School of Medicine, Denver, CO, USA Pulmonary hypertension (PHT) is a progressive disease that carries high morbidity and mortality in children [1]. The predominant etiologies of PHT in children are idiopathic pulmonary arterial hypertension, pulmonary arterial hypertension associated with congenital heart disease, and developmental lung disease [2]. The diagnostic classification of PHT was recently updated at the Sixth World Symposium on PHT (WSPH) in Nice, France (2018). This clinical classification (Table 46.1) identifies five categories of disorders that cause PHT, with each group sharing similar hemodynamic, pathologic, and management strategies [2,3]. Although cardiac catheterization is used to define PHT, echocardiography is the most important noninvasive tool used in screening, diagnosing, and managing children with PHT [4–9]. Echocardiography provides the morphologic evaluation of the heart, noninvasive hemodynamic assessment via Doppler echocardiography, and functional assessment of both ventricles [5–7,10–12]. In this chapter, conventional and advanced echocardiographic imaging to assess pediatric patients with PHT is described. The right atrium (RA) functions as a reservoir for systemic venous return when the tricuspid valve is closed, a passive conduit during early diastole, and an active conduit in late diastole [13]. It is dilated in patients with PHT and represents decreased right ventricular (RV) compliance and RV diastolic dysfunction. Imaging of the RA is easily obtained in the apical four‐chamber view where RA dimensions of minor and major axis are measured and planimetry of the RA area in end‐systole is performed to evaluate for RA dilation (Figure 46.1) [11]. Indexed RA area to body surface area in adult patients with idiopathic pulmonary arterial hypertension has been a predictor of mortality and has been shown to be a prognostic marker for follow‐up of PHT patients in adults and children [14–16]. Normal right atrial sizes based on body surface area in children have been established [17]. Rising RA pressure in PHT patients results in dilation of the inferior vena cava (IVC). The subxiphoid sagittal view shows the IVC entering the RA and IVC diameter can be measured. In adults, the RA pressure can be estimated by IVC diameter and the presence of inspiratory collapse [18–20]. The IVC diameter ≤2.1 cm that collapses >50% with a sniff suggests a normal RA pressure of 3 mmHg (range, 0–5 mmHg). An IVC diameter ≥2.1 cm that collapses <50% with a sniff suggests a high RA pressure of 15 mmHg (range 10–20 mmHg) [11,19,21,22]. In children, the IVC diameter varies with age of the patient and the sniff test does not really work in children because the IVC is very distensible. Acute increase in RV pressure results in RV dilation as a compensatory mechanism to maintain RV output. Chronic increase in RV pressure causes RV remodeling with variable degrees of hypertrophy and dilation. Because of the complex geometric shape of the RV, 2D imaging requires multiple parasternal, apical, and subxiphoid views to evaluate the RV dimensions. Current guidelines recommend the inclusion of standardized imaging of the RV linear dimensions to evaluate for RV dilation [11]. The “RV‐focused view” is recommended to measure the RV size; it can be measured from the apical four‐chamber view at end‐diastole [11,23]. The basal diameter is measured at the level of the tricuspid valve, and the mid‐cavity diameter is measured at the middle third of the RV at the level of the left ventricular (LV) papillary muscle. The longitudinal dimension is measured from the plane of the tricuspid valve annulus to the RV apex (Figure 46.2). Normal reference values and z‐scores of the RV in pediatrics have been published [24,25]. RV dilation is an early sign of RV maladaptation to increased pressure overload and an early sign of RV dysfunction [26]. RV end‐systolic base/apex ratio is decreased in children with PHT compared with controls and is inversely correlated with echocardiographic and hemodynamic indicators of increased RV pressure afterload, suggesting that this ratio can reflect disease severity [27]. Table 46.1 Updated clinical classification of pulmonary hypertension Source: Adapted from Rosenzweig EB, Abman SH, Adatia I, et al. Paediatric pulmonary arterial hypertension: updates on definition, classification, diagnostics and management. Eur Respir J 2019;53:1801916. PCH, pulmonary capillary hemangiomatosis; PVOD, pulmonary veno‐occlusive disease. The interventricular septum (IVS) is evaluated in the parasternal short‐axis view and is an indirect measure of elevated RV pressure. RV pressure overload causes flattening of the IVS in end‐systole into the left ventricle (LV), resulting in a “D‐shaped” LV. The eccentricity index (Figure 46.3) is the ratio between the LV anteroposterior dimension and the lateral dimension at the level of the papillary muscle [28]. LV deformation of the interventricular septum is greatest in end‐systole in patients with RV pressure overload. An eccentricity index greater than 1 is abnormal and has been shown to correlate well with invasive measurements of pulmonary artery (PA) pressure and be associated with adverse clinical outcome in adults with PHT [15,29]. Serial evaluation of the eccentricity index with improvement in this index has been shown in targeted PHT therapy in adults [30]. The eccentricity index in children is worse in patients with idiopathic PHT compared with PHT associated with congenital heart disease [16]. Flattening of the IVS can be classified as mild, moderate, or severe depending on the degree of PHT. In the absence of tricuspid regurgitation (TR) to estimate RV pressure, septal flattening offers indirect evidence of elevated pressure. End‐systolic flattening of the IVS is a sensitive marker for RV systolic hypertension in children [31]. The RV/LV ratio (Figure 46.4) at end‐systole measured at the level of the papillary muscles incorporates RV dimension in the parasternal short‐axis view and has been shown to correlate with invasive measures of hemodynamics [32]. An RV/LV end‐systolic ratio greater than 1 is associated with adverse clinical outcomes in children with PHT [32]. Flattening of the IVS impairs LV filling and can result in decreased LV cardiac output. Figure 46.1 Apical four‐chamber view measurement of right atrial dimensions (major and minor axis) and right atrial area in end‐systole in a pulmonary hypertension patient. LA, left atrium; LV, left ventricle; RA, right atrium; RV, right ventricle. Figure 46.2 Measurement of right ventricular (RV) dimensions in end‐diastole with basal dimension at the level of the tricuspid valve (magenta line), the mid‐cavity dimension (blue line), and longitudinal dimension (orange line). LA, left atrium; LV, left ventricle; RA, right atrium. Figure 46.3 The eccentricity index can be measured from the parasternal short‐axis view. The eccentricity index is D2/D1 in a patient with severe pulmonary hypertension. Figure 46.4 Parasternal short‐axis view of the right and left ventricles at the level of the papillary muscles. The right ventricle‐to‐left ventricle (RV/LV) ratio is derived from RV diameter (magenta line) and LV (yellow line) diameter at end‐systole. An atrial level shunt in PHT provides relief of symptoms of severe PHT by increasing systemic flow via a right‐to‐left shunt, reducing RV preload and increasing cardiac output. Atrial level shunts are assessed in the parasternal short‐axis, subxiphoid short‐axis, and subxiphoid long‐axis views. Contrast echocardiography can be used to assess the patency of an atrial level shunt. Atrial septostomy improves symptoms and quality of life in children with severe PHT [33]. Some series advocate use of an atrial septostomy to prolong survival [34,35]. Pericardial effusion is associated with increased risk of a poor outcome in adult PHT patients but has not been shown to be a prognostic indicator for children [15]. The size of the pericardial effusion is not predictive of outcome. Pericardial thickening, presence of pericardial effusion, and thickness of the anterior pericardial recess are defined as the total pericardial score to score patients with PHT [36]. Increased pericardial thickening and increased pericardial effusion were found to be higher in adults with severe PHT. Tricuspid valve regurgitation provides the most consistent means for measuring RV and systolic pressure, in the absence of RV outflow tract obstruction [37]. Normally, TR has a maximal velocity of <2.5 m/s [38]. The normal estimated systolic pulmonary artery pressure (SPAP) is ≤35 mmHg [39]. SPAP can be estimated from a peak TR velocity by continuous‐wave Doppler using the modified Bernoulli equation [40]. This is the most useful noninvasive method to predict SPAP. The mean RA pressure is added to the result of the Bernoulli equation to determine the RV systolic pressure (RVSP). In the absence of RV outflow tract obstruction, the SPAP equals the RVSP. The equation is: Estimation of SPAP from the TR jet is dependent on the angle and the presence of a sufficient Doppler envelope (Figure 46.5). It is recommended that the TR velocity is obtained from multiple views (apical four‐chamber view or parasternal views) until the best Doppler angle and the maximal velocity are obtained. Studies in adults have shown that this method of estimating SPAP correlates linearly with hemodynamic assessment in cardiac catheterizations [37,41]. In pediatric patients with chronic lung disease, estimation of SPAP using TR velocity has been shown to correctly diagnose the presence or absence of PHT in 79% of children but was only able to diagnose correctly the severity of PHT in 47% [42]. In a prospective trial of pediatric PHT patients using TR velocity to estimate SPAP compared with right heart catheterization, overestimation and underestimation of RV pressure occurred and TR velocity was inaccurate in children with elevated right heart pressures [43]. Nevertheless, TR velocity is still used clinically as a follow‐up tool to evaluate children with PHT. It is important to remember that a left‐to‐right shunt in ventricular septal defect (VSD) may contaminate the TR jet, which can result in erroneous RV pressure estimation. Figure 46.5 Tricuspid regurgitation (TR) estimating right ventricular pressure in a patient with pulmonary hypertension. The systolic (S) to diastolic (D) ratio from tricuspid regurgitation velocity can be measured to calculate the S/D ratio. The second method to evaluate SPAP is to use the VSD velocity (Figure 46.6a). In the absence of RV or LV outflow tract obstruction, the flow across the VSD can estimate the SPAP. This method has been shown to correlate well with invasive measurements obtained by cardiac catheterization [44,45]. The equation is: The maximal velocity across the ventricular septal defect is V(VSD)max and is the pressure difference between the two ventricles. A right‐to‐left shunt across the VSD and a low‐velocity left‐to‐right shunt suggests the presence of elevated pulmonary pressure. Parasternal and subxiphoid long‐axis views are used to interrogate membranous VSDs whereas parasternal and subxiphoid short‐axis views are best used to interrogate muscular VSDs. Maintaining a parallel angle between the VSD jet and Doppler beam is important to prevent underestimation of the true pressure drop between the ventricles. This is aided by using color Doppler and multiple views to obtain the maximum velocity. Muscular VSDs frequently pose a challenge, as it may be difficult to obtain a Doppler beam parallel to the jet, resulting in underestimation. Muscular VSDs are frequently long and irregular, making measurements potentially inaccurate. Unlike membranous and subarterial defects, where the diameter remains fairly constant throughout systole, those in the muscular septum frequently become smaller throughout the cardiac cycle, which will affect the velocity measurements. Lastly, SPAP can be estimated in patients with persistent ductus arteriosus (PDA) using the following equations: The maximal velocity across the PDA is denoted as V(PDA)max. This method has been validated by invasive measurements in cardiac catheterization [46]. The flow and velocity across the PDA are dependent on the pressure between the aorta and the main pulmonary artery. Right‐to‐left shunting across the PDA indicates that SPAP is higher than the aortic pressure (Figure 46.6b). Left‐to‐right shunting across the PDA indicates lower SPAP compared with the aortic pressure. Bidirectional shunting across the PDA is a common finding in newborns until the pulmonary vascular resistance has dropped (Figure 46.6b). The technique of using parallel Doppler interrogation applies to PDA velocity as well. If the angle of interrogation is difficult, if the PDA is very small, or if the PDA is very tortuous, then the pressure across the PDA can be underestimated or unreliable. The peak early diastolic velocity from pulmonary regurgitation can be used to estimate the mean pressure (mPAP). mPAP can be estimated from the equation (Figure 46.7): This equation has been shown to correlate well with invasive measurements in adults and children [47,48]. Pulmonary regurgitation velocity can be obtained in the parasternal short‐axis and subxiphoid right anterior oblique views. In adult studies, mPAP can also be estimated by using pulmonary acceleration time (AT) measured by pulsed‐wave Doppler of the pulmonary artery in systole, where mPAP = 79 – (0.45 × AT); in patients with AT <120 ms, the formula for mPAP = 90 ‐ (0.62 × AT) was better [49,50]. A newer method of evaluating mPAP is to add the mean RA pressure to the velocity–time integral of the TR jet. This method has been validated by invasive measurements in adults where the mean difference between mPAP calculated using this method was closer to the right heart catheterization mPAP [50–52]. These equations need validation in children. Figure 46.6 (a) Spectral Doppler pattern across a ventricular septal defect with low‐velocity left‐to‐right shunt, indicating pulmonary hypertension. (b) Spectral Doppler pattern across a patent ductus arteriosus with bidirectional shunt and low velocity, indicating pulmonary hypertension. The diastolic pressure (DPAP) can be estimated from the velocity of the end‐diastolic pulmonary regurgitant velocity using the modified Bernoulli equation (Figure 46.7): Figure 46.7 Spectral Doppler showing pulmonary regurgitation (PR) with peak early diastolic velocity and end‐diastolic velocity. Pulmonary vascular resistance (PVR) is calculated from cardiac catheterization as the pressure gradient across the pulmonary vascular bed divided by the pulmonary blood flow. PVR is important in the evaluation of PHT and can be estimated from echocardiography using the equation: where the VTI(RVOT) is the velocity–time integral of the RV outflow tract that can be obtained by spectral Doppler in the parasternal short‐axis view. In adults, this echocardiography derived PVR has been validated with cardiac catheterization [53]. In a study done in adults and children, PVR could be estimated using a simple ratio of peak TR velocity to the VTI(RVOT), and a value of >38 provided a specificity of 100% for a PVR of >8 Wood units (WU) [54]. However, another study using this equation in patients with very high PVR found that it did not correlate with invasive hemodynamic measurements [55]. In children with congenital heart disease, the ratio of isovolumic time (IVRT) to RV ejection time (ET) has been shown to correlate with PVR, with IVRT/ET <0.3 being 97% specific for a PVR <3 WU and a IVRT/ET ratio >0.4 highly predictive of PVR >5 WU [56]. Pande et al. used the TR velocity over VTI(RVOT) ratio (TR velocity/VTI(RVOT)) to correlate with invasive measurements of PVR in children with PHT in congenital heart disease [57]. The TR velocity/VTI(RVOT) ratio correlated well with PVR measured at catheterization. They found that for a PVR of 6 WU, a TR velocity/VTI(RVOT) value of 0.14 provided a sensitivity of 97% and a specificity of 93%, and for a PVR of 8 WU, a TRV/VTI(RVOT) value of 0.17 provided a sensitivity of 79% and a specificity of 95% [57]. Although echocardiography can estimate PVR, cardiac catheterization remains the gold standard in diagnosing PVR. Pulmonary vascular resistance reflects the resistance in the small vessels and the pulmonary vascular cross‐sectional area but does not account for the large and medium sized vessels or the pulsatile elements in the pulmonary circulation. Pulmonary vascular capacitance is a measure of workload on the RV. When the capacitance is low, all of the forward blood goes to the peripheral vessels, whereas when it is high the blood is initially stored in the large‐capacitance vessels, which decreases the load on the heart. A wide pulse pressure, seen in those with low capacitance, is a known risk factor for the left side of the heart [58]. The workload on a pump is proportional to forward output and the impedance of the vascular bed. Impedance is proportional to resistance and inversely proportional to capacitance. Pulmonary compliance represents the stretch of the vessel walls and how much they will increase in volume for a given pressure. Pulmonary vascular capacitance measures how much the vascular tree dilates with each contraction of the RV. Therefore, capacitance = stroke volume (mL)/pulse pressure (mmHg). By echocardiography, the stroke volume can be calculated from the left or right ventricular outflow tracts, for example: The pulse pressure is the peak systolic gradient from TR minus the end‐diastolic pressure from pulmonary regurgitation (PR), that is: where SV is stroke volume. This noninvasive measurement of pulmonary vascular capacitance was found to be the best predictor of adverse events in a group of adults with PHT, with a risk ratio of 3 : 1 [58]. This measurement was also a better predictor of adverse events than systolic, diastolic, and mean pressures; however, in a multivariate model pulmonary vascular resistance added to prognostic value. The measurement of pulmonary vascular capacitance is probably a strong predictor of adverse events as it takes into consideration the load on the right side of the heart and the status of the lung parenchyma. To measure pulmonary vascular capacitance, it is necessary to have a measurement of pulmonary diastolic pressure from pulmonary valve regurgitation. As not all patients have pulmonary valve regurgitation, other authors have used data derived from invasive techniques. This is based on the relationship between PA diastolic and systolic pressures [59]: Noninvasive PA capacitance can then be calculated based on this equation to obtain the pulse pressure and has been demonstrated to correlate well with invasive techniques in children [59]. Sajan et al. have demonstrated that invasively derived low PA capacitance index and high pulmonary vascular resistance index were independently associated with survival in children with PHT [60]. Takatsuki et al. demonstrated that pulmonary arterial capacitance index is a strong predictor of adverse outcomes in children with idiopathic and heritable PHT [61]. Pulmonary vascular resistance is a steady‐state parameter and measures opposition to continuous flow. However, this ignores the very nature of pulmonary flow, which is pulsatile. Flow into the pulmonary vascular bed requires a coordinated effort from the RV pump in systole and the pulmonary vascular bed in diastole. In an attempt to measure this variation throughout the cardiac cycle, a combination of change in PA luminal diameter throughout the cardiac cycle using color M‐mode Doppler tissue imaging (Figure 46.8), coupled with systolic pressure as measured from TR, has been used [62]. The use of color M‐mode with Doppler tissue imaging has higher spatial (1.6–1.9 mm) and temporal resolution (5 ms) than conventional echocardiography and allows for detection of instantaneous changes in the vessel diameter over time. There appears to be a very good correlation between the changes in diameter of the PA as measured by this technique compared with intravascular ultrasound: where D(s) is systolic diameter, D(d) is diastolic diameter, and P(s) is systolic pressure. This technique may play a role in evaluating treatment regimens for PHT. For example, increased PA pressure may produce a stiffer vessel on the basis of the strain‐stiffness effect, rather than being secondary to abnormalities of the vessel wall. With vasodilator therapy, if it is a strain‐stiffness effect then the response to treatment will be different than if there is a true abnormality in the vessel wall. In one study it was found that a >40% change in RPA diameter per 100 mmHg was consistent with a compliant vessel, whereas a value of <40% indicated a stiffer vessel [62]. This technique is not applicable if there is significant pulmonary regurgitation. Of note, the authors commented that the 40% cut‐off value may change through further studies in which a wider variation of pressures could be assessed. In addition, the technique required further validation with high pressure‐high flow and high pressure‐low flow situations. Figure 46.8 Color M‐mode Doppler tissue imaging demonstrating the luminal diameter of the right pulmonary artery (RPA). Time intervals can also be used as indirect techniques for the assessment of PA pressure [63]. Most of the studies validating this methodology have been performed in adults, with slower and less variable heart rates. Almost every time interval is intrinsically influenced by heart rate, which is problematic in the pediatric population and has been difficult to use in pediatric studies. Different time intervals can be measured. Pre‐ejection period (PEEP) is measured from the onset of the “q” wave to the onset of the PA systolic forward flow. The duration of PEEP is directly related to the PA pressure and pulmonary arterial impedance but is inversely proportional to RV contractile function. Pulmonary ejection time (ET) measures the duration of pulmonary forward flow during systole. With rising PA pressure, the spectral Doppler pattern changes from a smooth round shape to a triangular shape [64]. As for PEEP, this is heart rate dependent. At a given heart rate, ET is directly related to stroke volume and inversely related to contractility. Acceleration time (AT) is measured as the time interval from the onset to the peak of ejection (Figure 46.9). AT is directly related to RV systolic function and is inversely related to PA pressure and PVR. Right ventricular AT is decreased in patients with PHT. A ratio of AT/ET <0.3 is suggestive of PHT but cannot reliably determine the severity of PHT. If AT is <100 ms in adults, this suggests the presence of PHT. Recent studies have demonstrated that PA AT (PAAT) is useful in providing a reliable estimate of invasive pulmonary hemodynamics [65]. This study demonstrated that for the detection of PHT, PAAT <90 ms and PAAT/ET <0.31, the sensitivity was 97% and specificity was 95% [65]. Normal values of PAAT have been established in preterm neonates to 1 year of corrected gestational age and PAAT increases with maturation [66]. If this value compared with the normal value is shorter, then PHT can be suspected. Normal reference values and z‐scores of PAAT in children have also been established and an abnormal PAAT value with a z‐score of less than –2 was predictive of PHT [67]. Figure 46.9 Spectral Doppler of the pulmonary artery with normal acceleration time and short acceleration time in pulmonary hypertension. AT, acceleration time, ET, ejection time. Systolic time interval from the TR jet can be measured. In PHT, the systolic time interval from the TR jet will be increased. Alkon et al. used a simple measure of systolic‐to‐diastolic time (S/D) ratio (see Figure 46.5) from the TR jet to evaluate pediatric PHT patients [68]. When RV function worsens, the systolic portion of the cardiac cycle lengthens, leading to an increased S/D ratio [68]. This ratio was found to be higher in pediatric PHT patients compared with controls and is associated with worse hemodynamics by cardiac catheterization, shorter 6‐minute walk test, and worse clinical outcomes independent of PVR or pressures [68]. An increased S/D ratio >1.4 inversely correlates with survival in pediatric PHT [68]. Systolic RV function is an important prognostic determinant of PHT, and the ability of the RV to function under increased afterload is an important determinant of illness severity. The echocardiographic assessment of RV function is described in detail in Chapter 9. Evaluation of RV systolic function by echocardiography is difficult because of its complex geometric shape [69]. The RV is a bipartite chamber comprised of a sinus, which includes the atrioventricular canal region at the base and a trabeculated apex, and an outflow represented by the infundibulum (see Chapter 3). The RV has inner longitudinal fibers that result in base‐to‐apex contraction and superficial circumferential muscle fibers responsible for its inward bellow movement [70]. The evaluation of RV systolic function can be divided into global and regional systolic function. The RV fractional area change (FAC) is obtained from the apical four‐chamber view and is a measure of RV systolic function. It is calculated by the following equation:
CHAPTER 46
Echocardiographic Assessment of Pulmonary Hypertension
Introduction
Conventional 2D echocardiography
Right atrium
Inferior vena cava dilation
Right ventricle
Interventricular septum
Atrial level shunt
Pericardial effusion
Hemodynamic assessment using Doppler echocardiography
Right ventricular and pulmonary artery pressure
Mean pulmonary artery pressure
Diastolic pulmonary artery pressure
Pulmonary vascular resistance
Pulmonary artery capacitance
Pulmonary arterial compliance
Systolic time intervals from Doppler echocardiography
Right ventricular systolic function in pulmonary hypertension
Global assessment of right ventricular function
Right ventricular fractional area change
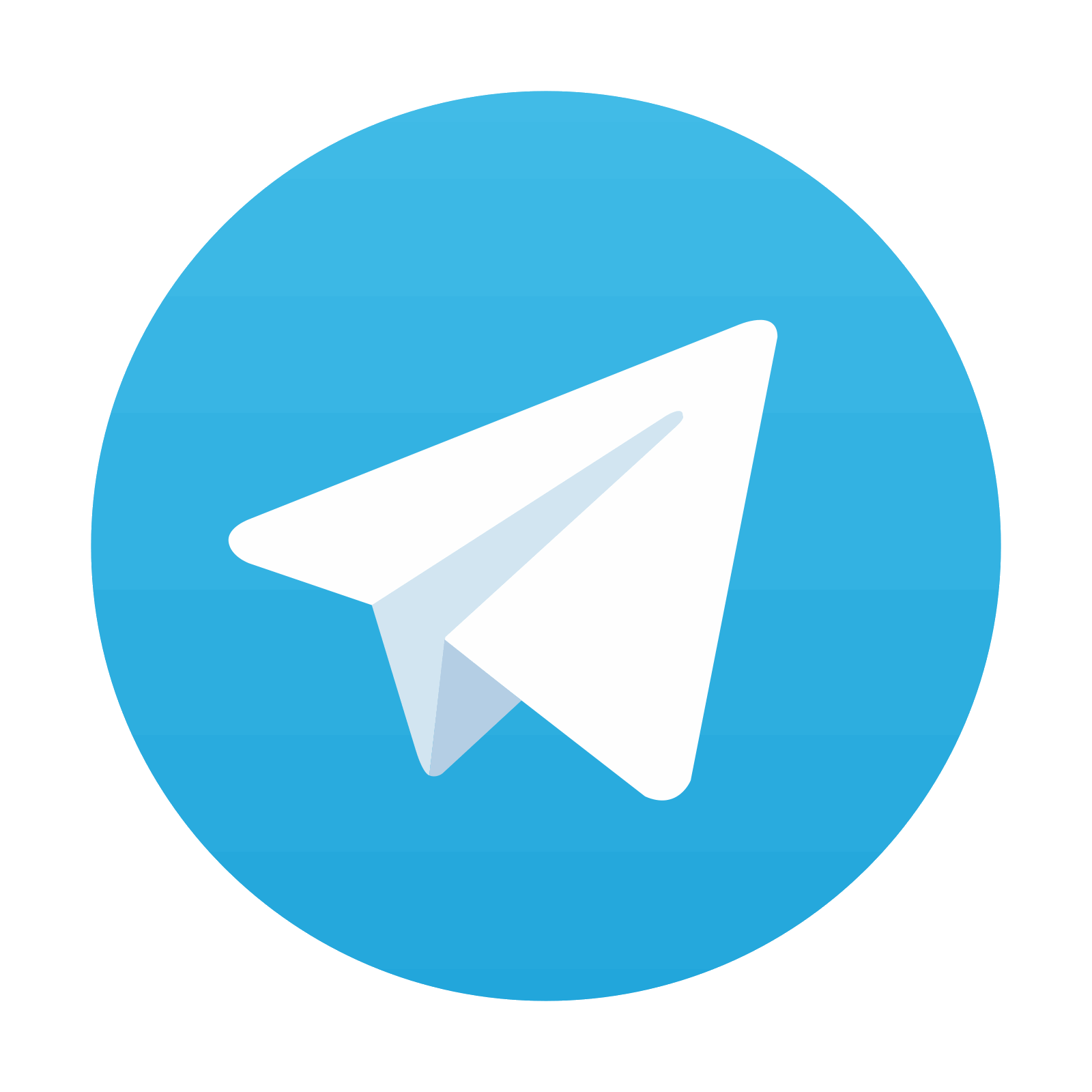
Stay updated, free articles. Join our Telegram channel
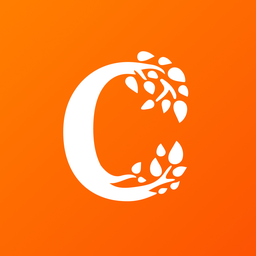
Full access? Get Clinical Tree
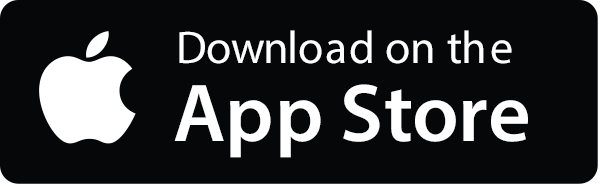
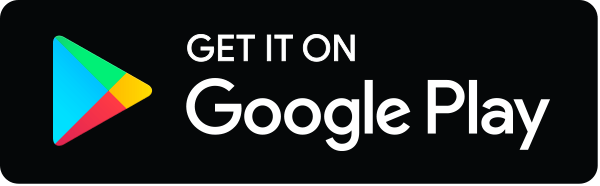