Matthew S. Lemler1, Poonam P. Thankavel2, and Claudio Ramaciotti1 1 University of Texas Southwestern; Children’s Medical Center of Dallas, Dallas, TX, USA 2 Medical City Children’s Hospital, Dallas, TX, USA Pulmonary valve stenosis is the fourth most common congenital heart lesion, occurring in approximately 53 (range 35–83) per 100,000 live births [1]. Valvar stenosis is virtually always congenital and usually found in isolation, although acquired cases due to carcinoid disease and rheumatic heart disease can occur [2]. In contrast, pulmonary regurgitation is primarily a complication of interventions to relieve valvar obstruction, although it can also be a consequence of valvar endocarditis or in rare cases of isolated absent pulmonary valve syndrome with intact ventricular septum. Presentation is determined by the degree of stenosis and/or regurgitation, with symptoms often related to hemodynamic effects on the right ventricle (RV). Timing of diagnosis and intervention can range from in utero or neonatal in severe cases to discovery in the older patient evaluated for an asymptomatic murmur. Importantly, dysplasia of the pulmonary valve is associated with an increased incidence of additional cardiac and noncardiac malformations [3]. Genetic syndromes that are commonly associated with a dysplastic pulmonary valve include Noonan syndrome, congenital rubella, neurofibromatosis, and multiple lentigines syndrome (LEOPARD syndrome), while Williams and Alagille syndromes primarily cause obstruction of the distal pulmonary arteries [4,5]. Familial occurrences of pulmonary valve stenosis have also been reported [6]. The normal pulmonary valve consists of three equal‐sized leaflets (right, left, anterior) supported in a semilunar fashion within the sinuses of the pulmonary trunk. The leaflets are made of a thin layer of endocardium and are separated from the tricuspid valve by a free‐standing infundibulum (conus). Superiorly, the leaflets are attached to the pulmonary trunk at the sinotubular junction while the basal portions are supported by the muscular infundibulum. It is this basal “ring” that is measured as the pulmonary “annulus” by echocardiography. The true anatomic junction between the muscular infundibulum and pulmonary trunk lies superior to this basal ring [7]. The free edges of each leaflet are appreciably longer than the chord of the sinus that supports it, which allows the leaflets to fit snugly together in the closed position [8]. Morphologic types of pulmonary valve stenosis can be divided into three categories: Post‐stenotic dilation of the main pulmonary artery (Figure 17.4) is common in patients with doming pulmonary valve stenosis and can rarely result in aneurysm formation [13], although it is not typically seen in the dysplastic pulmonary valve subtype [6]. Dilation usually extends into the left pulmonary artery but the right pulmonary artery may be involved as well; this can be attributed to a higher pressure in the left pulmonary artery compared with the right [14]. The degree of stenosis does not correlate with the size of the main pulmonary artery. The dilation is thought to be related to the eccentricity of the high‐pressure jet causing mechanical stress on the wall of the main pulmonary artery which has low resistance and high compliance [15]. Speculation continues to exist that dilation might be caused by an arteriopathy; however, this has not been confirmed. Figure 17.1 Parasternal long‐axis view tilted anterior towards the dysplastic, doming pulmonary valve. Ao, aorta; LA, left atrium; MPA, main pulmonary artery; RA, right atrium. Figure 17.2 Parasternal short‐axis view of a dysplastic pulmonary valve. MPA, main pulmonary artery; RVOT, right ventricular outflow tract. Figure 17.3 High left parasternal image of a quadricuspid pulmonary valve. Figure 17.4 Parasternal short‐axis view demonstrating a mildly doming and thickened stenotic pulmonary valve with significant dilation of the main pulmonary artery (MPA). RVOT, right ventricular outflow tract. The clinical consequences of pulmonary valve stenosis are typically related to the degree of obstruction and the effect on the RV. Progression of pulmonary valve stenosis is common in the neonatal period, in part due to normal hemodynamic changes, including increased cardiac output [16], decreased pulmonary vascular resistance [17], and closure of the patent ductus arteriosus (PDA). The rate of progression tapers off during infancy and is uncommon beyond the first year of life. Normal or only mild right ventricular hypertrophy occurs in response to mild or moderate pulmonary valve stenosis. Chronic pulmonary stenosis leads to right ventricular hypertrophy, especially in the outflow tract or infundibulum. A hypertrophied RV may maintain its function for years [18]; however, when severe right ventricular hypertrophy occurs, there is reduced end‐systolic deformation of the RV, diminished elastic recoil, and elevated RV pressure in early diastole [19]. Significant elevation of RV diastolic pressures results in elevation of right atrial pressure, poor RV compliance, and dilation of the right atrium. Right‐to‐left shunting may then occur in the presence of an atrial septal defect (ASD) or a patent foramen ovale (PFO) (Figure 17.5). Mahfouz et al. demonstrated abnormal systolic and diastolic parameters in children with moderate to severe pulmonary valve stenosis, including mitral annular plane excursion, tricuspid annular plane excursion, left ventricular systolic wave, right ventricular systolic wave, and myocardial dysynchrony. All parameters were significantly improved after pulmonary valvuloplasty [20]. Figure 17.5 Subxiphoid long‐axis (frontal) view demonstrating a right‐to‐left (R‐L) atrial shunt across the patent foramen ovale (arrow) in a patient with critical pulmonic valve stenosis. LA, left atrium; RA, right atrium. Both severe and critical pulmonary valve stenosis in a neonate will result in systemic or suprasystemic RV pressures. Critical pulmonary stenosis occurs when the RV is unable to maintain systemic arterial saturations above 90% in the absence of a PDA. When the RV is unable to eject the entire systemic venous return across the pulmonary valve, deoxygenated blood crosses the PFO into the left atrium causing systemic arterial desaturation [21]. Adequate pulmonary blood flow then becomes dependent on left‐to‐right shunting across the PDA. This causes a volume load on the left ventricle (LV), which is already exposed to the increased afterload that occurs during the neonatal transitional period. Poor biventricular function ensues. Marked right ventricular hypertrophy develops because of increased wall stress associated with severe stenosis. Infundibular hypertrophy may lead to anatomic and/or dynamic subvalvar obstruction. This dynamic obstruction can increase significantly immediately after balloon valvuloplasty, resulting in reduced cardiac output; hence assessment of the right ventricular outflow tract after the procedure is an important part of cardiac catheterization (Figure 17.6) [22]. When critical pulmonary valve stenosis occurs in the presence of severe tricuspid valve regurgitation, the RV suffers from both a pressure and volume overload, resulting in increased right‐to‐left atrial shunting. It is important to differentiate organic pulmonary valve obstruction from functional pulmonary valve atresia in neonates. The latter is defined as a structurally normal pulmonary valve that does not open in systole due to high postnatal pulmonary vascular resistance and an inability of the RV to eject. It is usually seen in the setting of severe tricuspid regurgitation (Ebstein anomaly, dysplastic tricuspid valve) and/or right ventricular myocardial abnormalities (Uhl anomaly) but has been reported rarely in neonates with normal intracardiac anatomy [23,24]. Accurate diagnosis is critical as the management pathways vastly differ. Echocardiography is vital in demonstrating subsystemic right ventricular systolic pressure estimates; continuous pulmonary insufficiency, when present, can be diagnostic although lack of pulmonary insufficiency does not rule out functional pulmonary atresia (Figure 17.7, Video 17.2) [25]. As the pulmonary vascular resistance decreases and RV function improves, the valves of a patient with functional pulmonary valve atresia will begin to open and the RV cardiac output will increase. Improved RV function and decreased pulmonary vascular resistance will have minimal effects on the cardiac output in patients with an abnormal valve, however the gradient across the valve will increase. Figure 17.6 Continuous‐wave Doppler placed just below the pulmonary valve demonstrating a “dagger” shape to the obstruction, which signifies dynamic obstruction of the right ventricular outflow tract immediately following balloon valvuloplasty of the valve. Pulmonary valve anatomy and physiology are well defined by 2D and Doppler imaging. The pulmonary valve is appreciated in the parasternal short‐axis view at the base of the heart (Figure 17.1, Video 17.3) and in the parasternal long‐axis view by tilting the transducer toward the patient’s left shoulder (Figure 17.8, Video 17.4). In addition to the morphology of the valve, the subxiphoid short‐axis and right anterior oblique views provide an excellent opportunity to assess additional levels of obstruction and further define the anatomy (Figure 17.9, Video 17.5). In infants and young children, the apical four‐chamber view can be adapted by sweeping anterior and clockwise to include the pulmonary valve and subvalve region (Video 17.6). Using standard echocardiographic views, the pulmonary valve is imaged in its longitudinal axis. However, using a high left parasternal view, a cross‐sectional image of the pulmonary valve can be obtained by visualizing the short axis of the aortic valve and rotating the transducer clockwise ~10–20° (Figure 17.10) [26]. The cross‐sectional view of the pulmonary valve can also be successfully performed with 3D imaging in about 60–70% of patients (Figure 17.11) [27]. 3D imaging has the potential to enhance the details of the pulmonary valve morphology; however the incremental benefit has yet to be established. It is important to assess the morphology and leaflet motion of the pulmonary valve. The smooth, domed valves typically respond well to balloon dilation resulting in the tearing/disruption of the fused commissures, while the dysplastic, trileaflet valves often require surgical intervention [28–30]. Careful inspection is necessary to differentiate between a doming pulmonary valve and discrete, supravalvar stenosis from a membrane or a narrowing at the sinotubular junction. In some cases, the distinction is difficult. Post‐stenotic dilation of the main and branch pulmonary arteries may be noted and should be measured; only rarely is the post‐stenotic dilation clinically relevant. An exception to this is absent pulmonary valve syndrome, where significantly dilated branch pulmonary arteries may result in airway compression. Prior to referring an infant to the cardiac catheterization laboratory, it is imperative to ensure the adequacy of both the pulmonary and tricuspid valves. Converting absolute measurements to z‐scores based on body surface area allows for standardized assessment of valve diameters. The tricuspid valve z‐score can be used as a surrogate for RV size and volume, since RV volumes are very difficult to quantify by echocardiography. Figure 17.7 Parasternal short‐axis view in color compare mode of an infant with functional pulmonary valve atresia. Note the pulmonary insufficiency jet that occurs during systole. On 2D imaging, note the well‐formed but closed leaflets of the pulmonary valve. Figure 17.8 Parasternal long‐axis view of a patient with mild pulmonary valve stenosis. LV, left ventricle; MPA, main pulmonary artery; RVOT, right ventricular outflow tract. Quantification of pulmonary valve stenosis is performed primarily by PW and CW Doppler. The estimated systolic pressure gradient is derived from the transpulmonary velocity flow curve using the simplified Bernoulli equation: The Bernoulli equation is most accurate in patients with discrete stenosis and becomes less reliable in patients with long‐segment stenosis or multiple levels of obstruction in series. Doppler will underestimate catheter‐derived gradients if there is a high divergence (>20%) between the ultrasound beam and the direction of blood flow [31]. To ensure accuracy it is important to line up the Doppler sample volume parallel to the direction of flow with the aid of color flow mapping. This must be performed in multiple views including the parasternal short‐axis, subxiphoid, and modified five‐chambered views [32]. Although Doppler‐derived peak instantaneous gradients are often higher than the peak‐to‐peak gradient measured in the cardiac catheterization laboratory, the literature supports the accuracy of Doppler‐derived gradients to predict catheter‐derived maximum instantaneous gradients [32–34]. In contrast, Silvilairat et al. reported that mean Doppler gradients more accurately predict catheter peak‐to‐peak gradients in both isolated pulmonary valve stenosis [35] and in complex pulmonary outflow stenosis [36]. Therefore, the authors of this chapter recommend measuring both peak instantaneous and mean gradients when assessing the severity of pulmonary valve stenosis. It is important to utilize PW Doppler to detect different levels of obstruction in the outflow tract. The pattern of the Doppler envelope can be helpful in identifying dynamic subvalvar obstruction, which has a typical “dagger” shape with peak obstruction occurring at end‐systole, in contrast to obstruction at the valvar level, where peak obstruction is in early systole [37]. Figure 17.9 Subxiphoid right anterior oblique view of a dysplastic pulmonary valve. Note the markedly thickened leaflets. Right ventricular hypertrophy is also seen. MPA, main pulmonary artery; RA, right atrium. Figure 17.10 High left parasternal image demonstrating a normal trileaflet pulmonary valve. Figure 17.11 Three‐dimensional image of the pulmonary valve from a transthoracic echocardiogram. The valve is seen en face from the view of the main pulmonary artery. When evaluating a patient with pulmonary valve stenosis by Doppler, it is important to understand that pressure gradients depend on both the effective valve orifice area and the flow rate across the valve. Conditions that reduce the flow across the valve will underestimate the degree of obstruction. This includes severe tricuspid valve regurgitation and RV dysfunction. Additionally, with pulmonary artery hypertension or a large PDA, the distal pulmonary artery pressure is elevated, thus masking the true impediment to flow. It is thus possible to have severe stenosis with little or no Doppler gradient. In fact, critical pulmonary valve stenosis can sometimes only be distinguished from pulmonary valve atresia by the presence of a trivial quantity of pulmonary regurgitation, when the RV is unable to eject blood across the dysplastic valve. Conversely, increased flow across the pulmonary valve in the setting of significant left‐to‐right intracardiac shunts may cause overestimation of gradients [38]. Figure 17.12 Transesophageal echocardiographic image with 84° rotation demonstrating a normal pulmonary valve. Ao, aorta; MPA, main pulmonary artery; RVOT, right ventricular outflow tract. Right ventricular hypertrophy and the degree of systolic flattening of the ventricular septum are additional semiquantitative methods to evaluate the degree of obstruction across the pulmonary valve. It is important to confirm RV systolic pressure estimates by measuring the tricuspid regurgitation velocity. The anterior location of the pulmonary valve and its proximity to the left upper lung lobe may limit the value of transesophageal echocardiography (TEE) in its visualization. The pulmonary valve and the right ventricular outflow tract may be imaged from a midesophageal window by rotating the transducer ~50–70° and from a deep transgastric window with a transducer angle of ~50–90° (Figure 17.12, Video 17.7) [39]. 3D TEE of the pulmonary valve is often limited but can be obtained (Figure 17.13, Videos 17.8 and 17.9). Gradients across the pulmonary valve are best obtained by rotating the transducer to 0°, maximally flexing the probe and turning slightly counterclockwise. Trivial to mild pulmonary valve regurgitation is common and can be considered a normal finding when accompanied by normal valve morphology. More severe regurgitation occurs with dysplastic valves, and most commonly after intervention. Acquired forms of pulmonary regurgitation are rare and include pulmonary artery hypertension, endocarditis, and carcinoid and rheumatic valvar disease [40]. Many studies analyzing the long‐term effects of pulmonary regurgitation have used either subjective or semiquantitative methods. Detection and grading severity are performed principally with color flow mapping and spectral Doppler. Methods include determination of overall jet size, vena contracta (VC), pressure half‐time, pulmonary regurgitation index, and presence of diastolic flow reversal in the branch pulmonary arteries [41,42] The maximum color jet width measured immediately below the pulmonary valve suffers from high intraobserver variability; however, a jet width that occupies >65% of the right ventricular outflow width is associated with severe pulmonary regurgitation [43]. The proximal jet width (VC) is more reproducible, and a ratio of the VC to the pulmonary annulus of >0.5 correlates well with severe pulmonary regurgitation defined as a regurgitant fraction of ≥40% by cardiac magnetic resonance (CMR). Optimization of the Nyquist scale in this measurement is crucial as the pulmonary regurgitation velocity is usually low in the setting of normal pulmonary artery pressures [42]. PW and CW Doppler can be used to measure the end‐diastolic pressure difference between the RV and the pulmonary artery. A “no flow time” (diastolic period – duration of pulmonary regurgitation) >80 ms and a pressure half‐time <100 ms has distinguished angiographic severe pulmonary regurgitation from milder forms of disease [44]. A pulmonary regurgitation index has been described as the ratio of pulmonary regurgitation duration by CW Doppler to total diastolic time. An index of <0.77 correlated well with a regurgitant fraction of >24.5% (>moderate) by CMR [45]; however, this ratio alone may overestimate pulmonary regurgitation in conditions where RV diastolic pressures are elevated and must be used in conjunction with secondary markers. Finally, the presence of diastolic flow reversal in the branch pulmonary arteries evaluated by color and Doppler is a sensitive indicator of severe pulmonary regurgitation as correlated by CMR in pediatric and adult patients with a history of isolated pulmonary stenosis or tetralogy of Fallot repair [46]. 3D imaging offers a potential, but still unproven, improvement in quantification of pulmonary regurgitation. 3D color Doppler allows the cropping plane to be positioned exactly parallel to the VC, which then can be planimetered and can be used to calculate the severity of pulmonary regurgitation [27]. Poor visualization of the pulmonary valve has limited the progress in developing 3D algorithms for quantification of pulmonary regurgitation [47]. Isolated pulmonary valve stenosis can be diagnosed in the fetus. In patients with mild or moderate pulmonary valve stenosis, the cardiac chambers tend to be normal in size. Additionally, the PDA demonstrates normal antegrade right‐to‐left flow in the fetus. Therefore, the examiner must rely on the size, morphology, and flow acceleration across the pulmonary valve [48]. Since the pulmonary valve is typically larger than the aortic valve at all stages of gestation, the finding of a pulmonary valve that is smaller than the aortic valve should raise suspicion for pulmonary valve stenosis even without an associated flow disturbance. Early in gestation, mild forms of pulmonary valve stenosis are very easily missed if suspicion is not high. Thus, pulmonary valve stenosis is often underdiagnosed in ultrasound performed prior to the third trimester. Figure 17.13 Three‐dimensional transesophageal echocardiographic image demonstrating a normal pulmonary valve en face. The leaflets are so thin that resolution is limited. In fetuses with severe or critical pulmonary valve stenosis, the morphology of the RV is determined by the degree of tricuspid valve regurgitation. The RV is dilated, and the ventricular wall is normal or thin in the presence of severe regurgitation, whereas in patients without tricuspid regurgitation, the RV is hypertrophied with various degrees of cavity hypoplasia (Figure 17.14). The degree of enlargement of the right atrium correlates with the degree of tricuspid valve regurgitation [49]. In the presence of tricuspid valve regurgitation, one must be careful to adequately inspect the tricuspid valve to exclude Ebstein anomaly or dysplasia of the tricuspid valve. In fetuses with critical pulmonary valve stenosis, flow across the PDA is retrograde from the aorta into the pulmonary arteries. This finding implies the need for intervention in the newborn period; however, antegrade flow across the PDA does not exclude a newborn from needing intervention [50]. Serial evaluations of the fetus diagnosed with pulmonary stenosis are important because progression from mild to critical pulmonary stenosis or even atresia has been well documented [49]. Fetuses with tricuspid regurgitation on early exams are at high risk of progressing to severe pulmonary stenosis or atresia. Alternatively, early increased flow velocity across the pulmonary valve (100–120 cm/s) at 14–17 weeks can resolve by around 22 weeks’ gestation [51]. It is important to assess flow across the PFO, as fetuses with severe pulmonary stenosis and PFO restriction are at high risk of developing hydrops fetalis [52]. As a result of decreased preload, the LV is unable to generate adequate cardiac output. Restrictive right‐to‐left shunting across the PFO is difficult to diagnose because the imager cannot rely on an increase in velocity to identify the restriction [53]. Therefore, it is imperative to look for signs of impending hydrops fetalis, including increased cardiothoracic ratio, pericardial effusion, holosystolic tricuspid valve regurgitation, and abnormal venous Doppler flow [52]. Figure 17.14 Fetal four‐chamber view in a patient diagnosed with critical pulmonary stenosis. A small hypertrophied right ventricle (RV) is noted along with a dilated right atrium (RA). LA, left atrium; LV, left ventricle. In the study of Gomez‐Montes and colleagues [54], a tricuspid‐to‐mitral valve diameter ratio of ≤0.83, pulmonary‐to‐aortic valve diameter ratio of ≤0.75, tricuspid inflow duration/cardiac cycle length of ≤36.5%, and RV‐to‐LV length ratio of ≤0.64 were associated with a univentricular repair after birth. Having three out of the four markers was 100% sensitive and 92% specific, whereas the presence of all four markers was 100% sensitive and specific in the second trimester of pregnancy. In addition to the tricuspid‐to‐mitral valve diameter ratio, tricuspid and pulmonary valve z‐scores were associated with a single ventricle outcome, but cut‐offs and reliability vary with gestational age [55]. Catheter balloon dilation is the primary modality for the treatment of doming pulmonary valve stenosis. Surgical intervention is reserved for patients with multiple levels of obstruction, dysplastic pulmonary valves, and markedly hypoplastic pulmonary valve annulus. While indications for balloon dilation of pulmonary valve stenosis remain controversial, the excellent results and low morbidity of the procedure have led to the use of balloon valvuloplasty even in patients with mild obstruction. Mullins has recommended balloon valvuloplasty for patients with Doppler‐measured peak instantaneous gradients as low as 35 mmHg if there are objective signs of right ventricular hypertrophy by echocardiography or electrocardiography [22]. Others feel that the RV systolic pressure should exceed 55–60 mmHg [8]. In the presence of severe tricuspid regurgitation or RV dysfunction, the gradient across the pulmonary valve cannot be relied upon to determine need for intervention. Echocardiography is the most important diagnostic modality for planning catheterization and surgical interventions. Measurement of the pulmonary annulus diameter is required to plan adequately for balloon sizing during the catheterization procedure. Typically, the balloon size used for valvuloplasty is 1.1–1.2 times the size of the pulmonary annulus, with a maximum balloon‐to‐annulus diameter ratio of 1.4. Echocardiography very closely approximates the angiographic measurement of the pulmonary annulus [56]. Since its original description in 1982, balloon valvuloplasty has become the treatment of choice for patients with congenital pulmonary valve stenosis [57]. Prior to this, surgical valvotomy was successful at relieving obstruction, but the need for pulmonary valve replacement due to pulmonary regurgitation was as high as 67% in a study with a median follow‐up of 33 years [58]. Transcatheter balloon valvuloplasty is also highly effective at reducing the gradient across the valve in up to 95% of cases [56,59,60], with significant pulmonary regurgitation occurring in 33% of patients in short‐term follow‐up [56]. Clinically, pulmonary regurgitation is mild in the first few months after valvuloplasty secondary to right ventricular hypertrophy and low compliance resulting in elevated end‐diastolic pressures; however, as RV compliance improves the pulmonary regurgitation may become more hemodynamically significant in the long term [61]. A recent multicenter study by Hansen et al. evaluated risk of residual stenosis (gradient >40 mmHg) and pulmonary regurgitation (≥ moderate) in 254 patients after balloon pulmonary valvuloplasty [62]. In multivariate analysis, smaller patients and higher pre‐procedural gradient were associated with the development of long‐term pulmonary regurgitation, while the presence of genetic abnormalities and higher pre‐procedural gradient had a higher incidence of residual obstruction with need for repeat intervention. Pulmonary valve replacement for residual pulmonary regurgitation was needed in only 1.7% of the cohort, but mean follow‐up was only 7.5 years [63]. In pulmonary stenosis requiring intervention, the role of echocardiography after the procedure is to identify complications such as perforation of the pulmonary valve leaflets or annulus, tricuspid valve injury, perforation of the right ventricular outflow tract, disruption of the pulmonary trunk, and/or cardiac tamponade [56]. In addition, the site and type of residual obstruction and severity of pulmonary regurgitation, if present, should be assessed. It is common for infundibular gradients to increase immediately after intervention; however, the gradient should improve during the first 18 months of follow‐up with remodeling of the hypertrophy [60]. In contrast, patients with residual valvar gradients do not improve [64]. Patients with dysplastic valves such as occurs with Noonan syndrome have higher residual gradients compared with nonsyndromic patients [62], leading some physicians to advocate for surgical repair in these cases. Consideration should be made for surgical repair of dysplastic valves especially when associated with a hypoplastic annulus and/or supravalvar obstruction. In patients with critical pulmonary valve stenosis with successful balloon angioplasty, 21% required additional procedures including repeat valvuloplasty, surgical enlargement of the right ventricular outflow tract, or a systemic‐to‐pulmonary arterial shunt or PDA stent [65]. Special care should be given to the echocardiographic assessment in these cases, with focus on the interatrial communication, PDA, and competency of the tricuspid valve. Most patients have adequate systemic oxygen saturations after balloon valvuloplasty. However, patients with hyperdynamic right ventricular outflow tracts can develop infundibular obstruction (so‐called “suicide” RV), causing inadequate flow across the right ventricular outflow tract. Other patients remain cyanotic secondary to right‐to‐left shunting across the PFO due to poor RV compliance or RV hypoplasia [66]. It is important to recognize these physiologic states by echocardiography, because both may require resumption of prostaglandin therapy. While the dynamic infundibular stenosis usually improves within several days, a poorly compliant RV may take several weeks to improve. In such cases, surgical creation of a systemic‐to‐pulmonary artery shunt or placement of a PDA stent should be entertained. In patients with a diminutive pulmonary annulus a systemic‐to‐pulmonary shunt may be required in addition to a transannular patch across the right ventricular outflow tract [67]. In the presence of significant pulmonary and tricuspid valvar regurgitation, there is a risk of blood flow regurgitating back into the RV, across the PFO into the left heart and back into the pulmonary artery via a PDA. This phenomenon is known as a “circular shunt” and may result in poor systemic cardiac output due to ineffective circulation. A circular shunt must be excluded in any patient with metabolic acidosis or cyanosis in the post‐interventional period. Subvalvular pulmonary stenosis includes infundibular pulmonary stenosis and double‐chambered right ventricle (DCRV). Infundibular stenosis can be primarily caused by a discrete fibromuscular obstruction or hypertrophic cardiomyopathy. Secondary forms include aneurysm of the ventricular septum, aneurysm of the sinus of Valsalva, accessory tricuspid valve tissue, cardiac tumors, and right ventricular hypertrophy secondary to pulmonary valve stenosis. Hypertrophic cardiomyopathy and the secondary forms will not be discussed in this chapter. Discrete fibromuscular subpulmonary obstruction is described as a ring or fibromuscular diaphragm with an orifice located at the infundibular ostium or within the RV infundibulum. DCRV encompasses conditions in which the muscular structures comprising the proximal infundibular ostium create a division within the RV, resulting in muscular narrowing between the RV sinus (inflow) and the infundibulum (outflow). Patients with this abnormality are also categorized as having anomalous RV muscle bundles. The progressive obstruction divides the RV into a proximal high‐pressure RV sinus chamber and a low‐pressure infundibular chamber distal to the muscular structures. The incidence of discrete fibromuscular obstruction has been reported to vary between 0.2% and 8% of all cases of pulmonary stenosis [68,69]. The incidence of DCRV is not accurately known. Due to its progressive nature, this lesion is more easily detected after the first months of life. It has been suggested that 3–7% of patients with a ventricular septal defect (VSD) will acquire pulmonary outflow obstruction [70,71]. Among all patients with a VSD seen at Boston Children’s Hospital between 1973 and 2002, 0.04% developed a DCRV [72]. Simpson et al. reported 10% prevalence in children undergoing VSD repair [73], and Moran et al. noted development of DCRV in 3.1% of patients undergoing tetralogy of Fallot repair before the age of 2 years [74]. In a series from Spain, 0.75% of all cardiac defects repaired in childhood included DCRV [75]. The embryologic mechanism suggested for the development of discrete fibromuscular obstruction is a defect in absorption of the anterolateral conus or pulmonary infundibulum [76]. There is no consensus about the origin of the anomalous muscle bundles that make up a DCRV. Some have suggested that the anomaly arises from high take‐off of the moderator band with acquired hypertrophy of the muscle bundles of the proximal os infundibulum (opening to the infundibulum), including septal, moderator, and parietal bands [77]. It has also been proposed that the anomaly is due to persistence of prominent septal–parietal trabeculations [78–82]. Possible relationships between DCRV and Down syndrome [83] and Noonan syndrome [84] have been proposed. In addition, Lougheed et al. have reported the development of right ventricular outflow tract obstruction in almost 10% of recipients of fetal twin‐to‐twin transfusion [85]. In its more common form, the obstruction caused by anomalous RV muscle bundles is not present in the first months of life. The muscle bundles become more prominent as the patient grows, and the obstruction is usually progressive [81]. However, rates of progression vary considerably [73,86]. In addition, several authors have described various forms of obstruction within the RV that are different from typical DCRV. These include an anomalous apical shelf with or without an associated Ebstein anomaly; an apical shelf confluent with the outlet septum that can give the impression of double‐outlet LV; a circumferential muscular diaphragm in the presence of tetralogy of Fallot; and more rarely an aberrant moderator band [87–90]. Subpulmonary obstruction caused by a discrete fibromuscular ridge usually begins at the lower portion of the infundibulum. RV cavity division by anomalous muscle bundles usually occurs at the junction between the sinus (inlet) and the infundibular (outlet) components of the RV. The anomalous muscle bundles have also been described to develop proximal to the usual right ventricular outflow obstruction found in tetralogy of Fallot [74]. It may also occur in patients with other conotruncal lesions such as D‐ or L‐loop transposition of the great arteries or double‐outlet RV [91]. Central perimembranous VSD is the cardiac anomaly most commonly associated with DCRV, although muscular and malalignment defects are also found. Another common association is between DCRV with or without a VSD and discrete subvalvar aortic stenosis [91]. The development of left ventricular outflow tract obstruction has been reported to occur before, concomitant with, or even years after surgical repair of DCRV. In the majority of patients, obstruction caused by anomalous muscle bundles is progressive [81], but rates of progression vary considerably [73,86]. Progressive right ventricular outflow obstruction causes an increase in the pressure gradient measured within the RV cavity. In most cases, the VSD communicates with the proximal high‐pressure RV sinus chamber [92]. The progression of obstruction in such patients causes a decrease in the amount of left‐to‐right shunting and a decrease in the Doppler pressure gradient from the LV to the RV. In the most severe cases of DCRV, the shunt across the VSD can reverse. Although some of these patients have been considered to have a form of tetralogy of Fallot, the two conditions are part of a continuum. However, spontaneous closure of the VSD and left ventricular outflow abnormalities are more frequently associated with DCRV whereas pulmonary annular hypoplasia, right aortic arch, and pulmonary artery abnormalities are typical in patients with tetralogy of Fallot. In patients with DCRV, the VSD may undergo spontaneous closure. It is widely believed that cases that present with an intact ventricular septum may actually have had spontaneous closure of a VSD. Right ventricular dysfunction, a late finding, is more commonly seen in patients with restrictive or even closed VSD and markedly elevated right ventricular hypertrophy and pressure.
CHAPTER 17
Anomalies of the Right Ventricular Outflow Tract and Pulmonary Valve
Pulmonary valve stenosis
Introduction and incidence
Morphology and classifications
Pathophysiology
Imaging
Echocardiographic assessment
Pulmonary valve regurgitation
Prenatal assessment
Transcatheter and surgical treatment
Post‐procedure assessment and long‐term sequelae
Subpulmonary stenosis, includingdouble‐chambered right ventricle
Introduction and incidence
Morphology and classification
Pathophysiology
Imaging
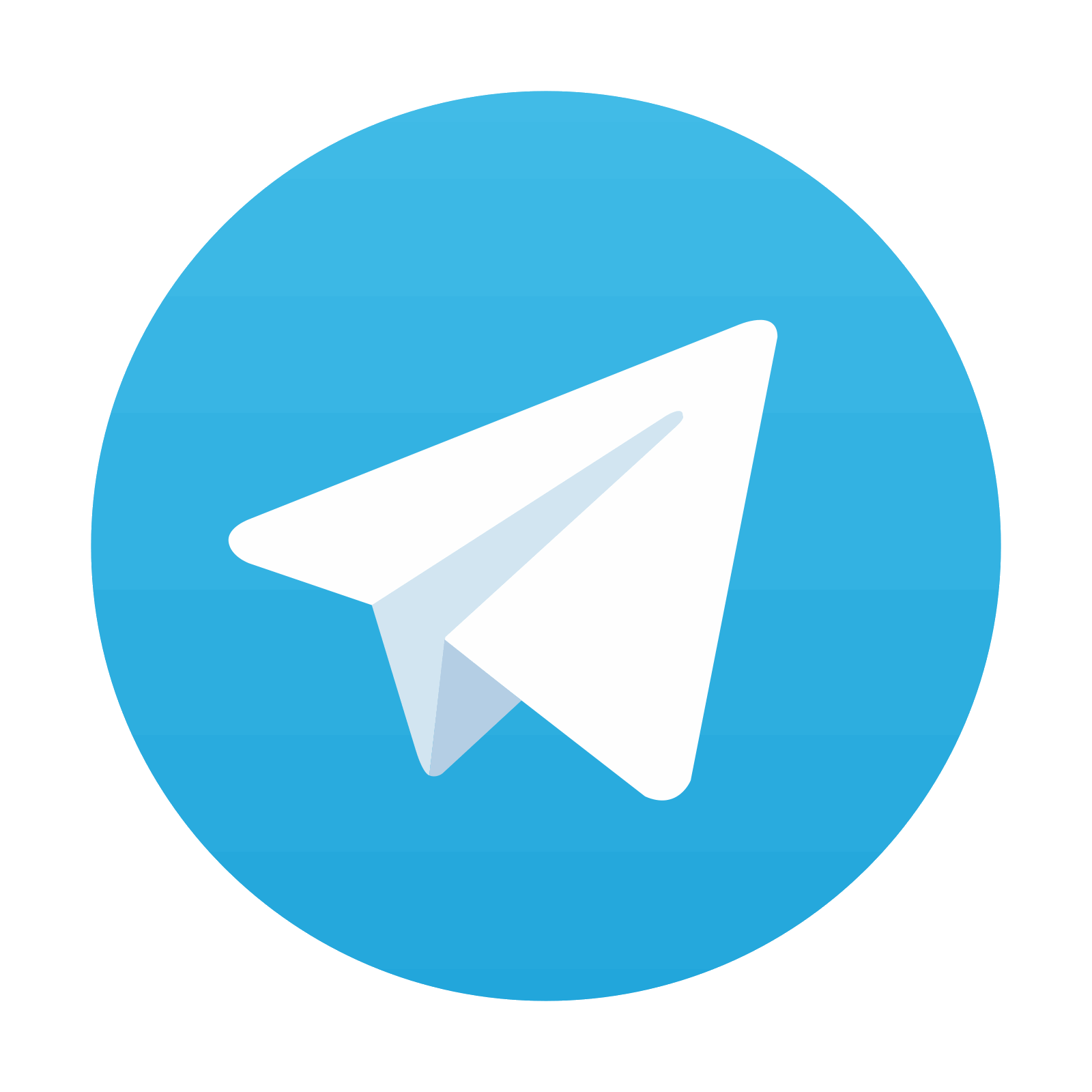
Stay updated, free articles. Join our Telegram channel
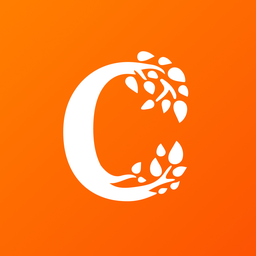
Full access? Get Clinical Tree
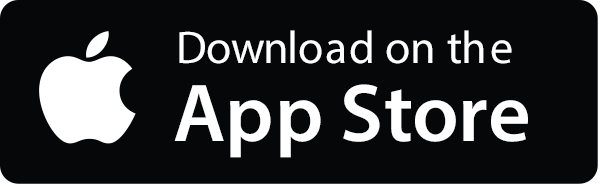
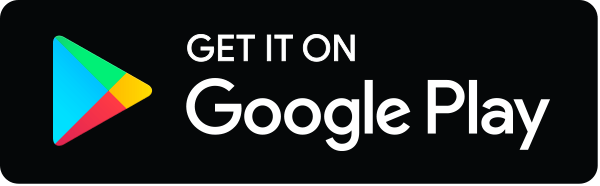