33 Mark G. Papich In 1928, Alexander Fleming observed that a Penicillium mold contaminating a Petri dish culture of staphylococci colonies was surrounded by a clear zone free of growth. Fleming cultured the contaminating mold on a special medium and demonstrated that the culture broth contained a potent antibacterial substance that was relatively nontoxic to animals, but was active against a variety of gram-positive organisms. He named the substance penicillin. In 1940, penicillin was isolated in the form of a brown, impure powder and was the most powerful chemotherapeutic agent known at that time. Since then, more than 40 penicillins have been identified. Some occur naturally; others are biosynthesized (semisynthetic penicillins). In 1945, Cephalosporium acremonium was isolated from raw sewage. The first cephalosporin, cephalosporin C, was derived from this fungus. All other cephalosporins are semisynthetic antibiotic derivatives of cephalosporin C. The first cephalosporin was available for clinical use in 1964. Since those first cephalosporins, they have developed through first, second, third, and fourth generations, all of which are used in veterinary medicine. There is now a commercially available fifth generation used in humans. The reader may notice differences in spelling. The older cephalosporins, derived from the fungus are spelled ceph, whereas the newer semisynthetic derivatives are spelled cef. Although penicillins, penicillin derivatives, and cephalosporins are still the most commonly used β-lactam antibiotics, much progress has been made in the development of new β-lactams during recent years. Most notably, these include the β-lactamase inhibitors (e.g., clavulanic acid), new cephalosporins, the carbapenems, also known as penems (e.g., imipenem, meropenem, ertapenem, doripenem), and the monobactams (e.g., aztreonam) (Abraham, 1987). β-lactam antibiotics exert their effects by preventing bacterial cell wall synthesis and disrupting bacterial cell wall integrity. These drugs are considered bactericidal in a time-dependent manner. They kill bacteria by inhibiting or weakening the cell wall. The cell wall of bacteria consists of alternating units of N-acetylglucosamine and N-acetylmuramic acid, which are cross-linked by short strands of peptides. A transpeptidation reaction is responsible for cross-linking the strands to form a strong, netlike structure. Inhibition of this transpeptidation reaction by acetylating the enzyme is one of the sites of action for β-lactam antibiotics. Interference with transpeptidation results in a weak cell wall and rupture of the bacteria. The binding sites for β-lactam antibiotics are called penicillin-binding proteins (PBPs), which are the enzymes that form the cell wall. There can be anywhere from two to eight distinct PBPs in bacteria, which are numbered according to their molecular weight. The most common PBPs affected by β-lactams are PBP-1, -2, or -3. Some of the variation in spectrum of action and bactericidal action of β-lactam antibiotics can be related to their relative affinity for different PBPs. For example, inhibition of PBP-1a and -1b generally cause lysis, PBP-2 results in rounded cells called spheroblasts, and PBP-3 produces long filamentous forms. The drugs that cause rapid lysis (for example, carbapenems) are the most bactericidal and have highest affinity for PBP-1. On the other hand, mutations in the enzyme, produced by the resistance gene mecA, produces PBP-2a, a penicillin-binding protein that resists binding of the β-lactam drugs and renders bacteria with this gene resistant. The PBP-2a target is the basis for resistance to methicillin and oxacillin, known as methicillin-resistant staphylococci (MRS) (Weese, 2005). In order to reach the site of action, these drugs first must penetrate the outer layer of the bacteria. They penetrate the outer layer of bacteria through a pore (a porin protein) that is ordinarily present in bacteria to allow nutrients to enter the cell. It is generally easier to reach the target site in gram-positive bacteria and they usually are more susceptible to the effects of β-lactam antibiotics than gram-negative bacteria. Gram-negative bacteria may have a thick outer membrane and have pores more difficult to penetrate. The drugs most effective against gram-negative bacteria are those that can rapidly penetrate the outer membrane of the cell wall. The β-lactam antibiotics are time dependent in their activity (Turnidge, 1998). β-lactam antibiotics are slowly bactericidal because of the slow rate of acetylation of the PBP, and the time of drug concentration above the minimum inhibitory concentration (MIC) (T > MIC) is important to clinical success (Drusano, 2004). At low exposure of β-lactams, not all of the available PBP are acetylated and bacterial stasis occurs (bacteriostatic effect). As the maximum number of PBP become acetylated, bactericidal activity occurs. Once maximum acetylation of the enzymes occurs the killing rate does not increase further, which explains the time-dependent effect of β-lactams, rather than a concentration-dependent effect. In some cases, drug concentrations can fall below the MIC for treating staphylococcal infections and still attain a cure because of a postantibiotic effect (PAE), but a PAE does not occur against gram-negative bacilli (Zhanel et al., 1991). Additionally, since the MICs are lower for gram-positive bacteria, longer dose intervals may be possible for infections caused by gram-positive as compared to gram-negative bacteria because it is easier to keep the plasma concentration above the MIC. The optimum duration of plasma concentrations above the MIC has varied among studies, but a general assumption is that the drug concentration should be above the MIC for 50% – and perhaps less – of the dosing interval (Turnidge, 1998; Drusano, 2004). This may vary depending on the immune competence of the animal and specific drug class. The carbapenem group of drugs (for example, imipenem and meropenem), are used with increasing popularity in small animal practice. These drugs are more bactericidal than penicillins and cephalosporins and the T > MIC for successful therapy may be less for these drugs than other β-lactams (for example, 30% of the dose interval). When immune competence is a question – for any of the β-lactams – increasing the time above MIC is advised. Dosage regimens for the β-lactam antibiotics should consider these pharmacodynamic relationships. Therefore, for treating a gram-negative infection, especially a serious one, it is necessary to administer many of the penicillin derivatives and cephalosporins three to four times per day if they have short half-lives. Some of the third-generation cephalosporins have long half-lives and less frequent regimens have been used for some of these drugs (e.g., ceftiofur–Naxcel, cefovecin–Convenia, and cefpodoxime proxetil–Simplicef). For highly susceptible gram-positive infections, less frequent administration may be acceptable. Note that the indications on the FDA-approved label for veterinary β-lactams may be aimed at these highly susceptible gram-positive pathogens and may not be appropriate for gram-negative bacteria with higher MIC values. Specific examples to illustrate this point are provided in this chapter. Three independent factors determine the bacterial susceptibility to β-lactam antibiotics: (i) production of β-lactamases, (ii) decreased penetration through the outer cell membrane to access the cell wall enzymes (which includes efflux pump mechanisms), and (iii) the resistance of the target (PBP) to binding by β-lactam agents (Gold and Moellering, 1996; Frere et al., 1991). An example of an altered target (PBP) is the one found in methicillin-resistant isolates, mentioned in Section Mechanism Of Action Of β-Lactam Antibiotics. The elaboration of β-lactamases, enzymes that inactivate the drugs by hydrolyzing the β-lactam ring, is the major mechanism of drug resistance (Jacoby and Munoz-Price, 2005). Bacteria produce β-lactamases that possess a range of physical, chemical, and functional properties. Some β-lactamases are specific for penicillins (penicillinases), some are specific for cephalosporins (cephalosporinases), and others have affinity for both groups. The genes that code for β-lactamases can occur via mutations in chromosomes, or they may be transferred by genetic elements. The genetic elements carrying these genes – which may be transferred among bacteria – include plasmids, which may be organized into integrons or carried on tranposons. A transposon may move (transpose) from DNA to plasmids, and vice versa. Integrons may be part of a transposon. The integrons may also contain genes that code for resistance to other drugs – thus producing multidrug resistance. There are many β-lactamase enzymes that are capable of hydrolyzing the cyclic amide bond of the β-lactam structure and inactivating the drug. Investigators have described over 190 unique β-lactamase proteins that have this ability. Classification schemes may vary, and β-lactamase enzymes have been categorized according to molecular structure and substrate, bacterial type (gram-negative versus gram-positive), transmission (plasmid coded versus chromosomal coded), and whether they are inducible or constitutive. The Ambler Class (Class A, B, C, and D), which uses amino acid sequencing, is now widely accepted (Rice and Bonomo, 2000; Jacoby and Munoz-Price, 2005). Class A enzymes are the most important in veterinary medicine. These include those listed in the following sections. These are produced by coagulase-positive Staphylococcus sp. and some coagulase-negative strains. The synthesis of these enzymes is determined by specific resistance genes and the enzymes are exocelluar. These enzymes typically do not inactivate cephalosporins and antistaphylococcal penicillins (e.g., isoxazolyl penicillins such as oxacillin or dicloxacillin). These β-lactamases can be inactivated by β-lactamase inhibitors (e.g., clavulanate acid, tazobactam, and sulbactam). This is a very diverse group that can arise through mutation or via transferable genetic elements. These enzymes are more wide spectrum and can hydrolyze penicillins (penicillinases), cephalosporins (cephalosporinases), or both. β-lactamases produced by Enterobacteriaceae such as E. coli belong to the TEM, SHV, and CTX-M, families, as well as other less common groups. Some, but not all, of these enzymes are inhibited by β-lactamase inhibitors (e.g., clavulanate, sulbactam). Gram-negative bacteria secrete small amounts of β-lactamases into their periplasmic space, allowing for optimal location of the enzyme to degrade the β-lactam upon entry into the organism. The advantage of newer drugs such as third-generation cephalosporins (also called oxyimino-β-lactams) and carbapenems is their ability to remain stable to gram-negative β-lactamases. Among the most serious of the gram-negative β-lactamases are the ESBLs – extended-spectrum β-lactamases. These β-lactamases will cause resistance to even the most active (extended-spectrum) cephalosporins. These enzymes are generally acquired through horizontal gene transfer (plasmids or transposons), but resistant clones also can spread in the local environment or hospital. Regardless of the mechanism, it presents a challenge to clinicians. Bacteria positive for ESBL can be difficult to treat because of limited drug options, and many are resistant to other classes of antibiotics. A more recently emerging β-lactamase resistance is the production of carbapenemase. This enzyme will inactivate the valuable carbepenem class of drugs. These bacteria have been identified as carbapenem-resistant Enterobacteriaceae (CRE), and include the Klebsiella pneumoniae carbapenemase (KPC). Bacteria that carry this resistance are very difficult to treat because there are so few available treatment options. Fortunately, this mode of resistance has not yet become a clinical problem in veterinary medicine, but there are concerns about its occurrence in animals (Abraham et al., 2014). Gram-negative bacteria can produce a cell wall with a modified outer membrane that is no longer permeable to β-lactam antibiotics. These impenetrable porin proteins can resist entry by β-lactam drugs. Reducing the permeability of the outer membrane limits antibiotic entry into the bacteria by down-regulation of the porin protein, or by replacement of the porin with a channel that resists entry by the drug. The porins can also delay or slow the rate of entry, thus making the drugs more susceptible to attack by β-lactamase enzymes. Therefore, this mechanism can enhance resistance produced by the elaboration of β-lactamases. Another mechanism that inhibits access to target sites is the efflux of β-lactams out of the bacteria. This mechanism actively transports the antibiotic out of the cell. When these pumps are expressed, it can produce a high level of resistance and can affect multiple drug classes – thus conferring multidrug resistance (MDR). The MDR efflux pumps may be carried on chromosomes or transferred by plasmids. Penicillin G is historically important because it was the first antibiotic introduced in medicine. Alexander Fleming discovered penicillin in 1928, but it was not used clinically until the early 1940s. Much of the early supply was designated for military use during World War II. It remains a popular antibiotic and is still the drug of choice for initial treatment of many infections in horses and cattle. It has little usefulness in small animals because of the high incidence of resistance. Penicillin is one of the few antibiotics that is still measured in terms of units rather than weight in mg or μg. One unit of penicillin represents the specific activity in 0.6 μg of sodium penicillin based on the International Standard for Penicillin. Thus, one mg of penicillin sodium represents 1,667 units of penicillin. Doses of other β-lactams are expressed in weight (mg) rather than units. Penicillin contains a fused ring system, the β-lactam thiazolidine (Figure 33.1). The physical and chemical properties, especially solubility, of penicillins are determined by the acyl side chain and the cations used to form salts. Figure 33.1 Structure of penicillins (left) and cephalosporins (right). Penicillins are composed of a five-member thiazolidine ring; cephalosporins are composed of a six-member dihydrothiazine ring. The structures can be modified into different drugs by replacing the R-groups or asterisk (*) with other functional groups. The most critical feature of the structure to exert its antibacterial action is the β-lactam ring. If the β-lactam ring is broken (hydrolyzed), the drug is inactive. Hydrolysis is the main cause of penicillin degradation, which can occur via bacterial enzymes (β-lactamases). This reaction also can occur from drug interactions (for example, in the syringe when penicillin is mixed with another drug). Some penicillins are poorly orally absorbed because they are unstable in the stomach and hydrolyzed by gastric acid. Penicillin is incompatible with heavy metal ions, oxidizing agents, and strong concentrations of alcohol. The penicillins are listed in categories based on their synthesis and spectrum of action. These include the natural penicillins (e.g. penicillin G), aminopenicillins (e.g. ampicillin, amoxicillin), antistaphylococcal penicillins (e.g. oxacillin), and the extended-spectrum penicillins (e.g. piperacillin). These are discussed in more detail in specific sections later in the chapter. The natural penicillins are active against many Streptococcus spp. and nonpenicillinase-producing Staphylococcus spp. They are active against some gram-positive, but only selected gram-negative bacteria, which include Arcanobacterium (formerly Actinomyces), Listeria monocytogenes, and Pasteurella multocida. Therefore, these drugs are narrow-spectrum drugs that are active against non–β-lactamase–producing gram-positive bacteria but few gram-negative bacteria. Many anaerobic bacteria are susceptible, including Fusobacterium, Peptococcus, Peptostreptococcus, and some strains of Bacteroides (except Bacteriodes fragilis group) and Clostridium. These drugs are also active against most spirochetes, including Leptospira and Borrelia burgdorferi. Natural penicillins are consistently inactive against Pseudomonas, most Enterobacteriaceae, and penicillinase-producing Staphylococcus spp. Aminopenicillins are active against the bacteria that are susceptible to penicillin G. They can penetrate through the outer membrane of gram-negative bacilli better than penicillin, which increases the spectrum to include some Enterobacteriaceae, including strains of E. coli, Proteus mirabilis, and Salmonella; however, most are resistant. Aminopenicillins are inactive against Pseudomonas, Bacteroides fragilis, and penicillinase-producing Staphylococcus spp. There are only subtle differences in antimicrobial activity between amoxicillin and ampicillin, and for susceptibility testing purposes, they are considered equivalent (CLSI, 2015). The antistaphylocccal penicillins include methicillin and nafcillin as well as the isoxazolylpenicillins (oxacillin, cloxacillin, and dicloxacillin). The isoxazolylpenicillins are considered as a group because of their structural similarity. These semisynthetic penicillins are active against many penicillinase-producing Staphylococcus spp. that would ordinarily be resistant to penicillin G and the aminopenicillins. They also have some activity against other gram-positive and gram-negative bacteria and spirochetes. They are rarely, if ever, used clinically except for intramammary products that contain cloxacillin. The extended-spectrum penicillins have the most activity against gram-negative aerobic and anaerobic bacteria of all of the penicillin groups. The drugs are active against many strains of Enterobacteriaceae and some strains of Pseudomonas. Carbenicillin and ticarcillin are active against some strains of E. coli, Morganella morganii, Proteus spp., and Salmonella. In addition to these organisms, mezlocillin and piperacillin are active against some strains of Enterobacter, Citrobacter, Klebsiella, and Serratia. These extended-spectrum penicillins have some activity against gram-positive aerobic and anaerobic bacteria but have no advantages against these organisms compared to penicillin G and aminopenicillins. Extended-spectrum penicillins are generally more active against Bacteroides fragilis than are other available penicillins. Of this group, few are available and used clinically. Ticarcillin and ticarcillin–clavulanate, once popular for injectable use, is no longer available. Carbenicillin is no longer available. Piperacillin–tazobactam is the most popular and widely available of this group. The CLSI has approved breakpoints for animals for most of the penicillins and derivatives (CLSI,). These breakpoints often reflect the accepted clinical use of the drug and not necessarily the approved label indication. For example, the breakpoint for procaine penicillin G was established using a dose of 22,000 U/kg IM, consistent with good clinical practice, rather than the FDA-approved label dose of 7,500 U/kg. CLSI approved breakpoints are listed in Table 33.1. Table 33.1 Susceptibility breakpoints for penicillin and derivatives (as approved by CLSI,). BRD, bovine respiratory disease; SRD, swine respiratory disease; PK, pharmacokinetic; PD, pharmacodynamic; R, resistant; I, intermediate; S, susceptible; UTI, urinary tract infection. Ampicillin and amoxicillin have been the most studied of the penicillin group and Table 33.2 lists the pharmacokinetic parameters in several domestic species. Penicillin G pharmacokinetics are presented in Table 33.3. When sodium salts of these drugs are injected, maximum blood concentrations occur usually within an hour. Table 33.2 Ampicillin and amoxicillin pharmacokinetics (mean values) Vd, volume of distribution; CL, systemic clearance; T½, half-life; Cmax, peak concentration; Tmax, time of peak concentration after absorption; F, fraction of dose absorbed. Table 33.3 Pharmacokinetic parameters for penicillin in animals IM, intramuscular; IV, intravenous; SC, subcutaneous; CMAX, peak concentration; TMAX, time of peak concentration after absorption. aCraigmill et al. (2004). Craigmill’s analysis used 18 published papers, 28 data sets, and 288 data points. They also reported a volume of distribution/ F of 13 l/kg (± 7.46). bMean values (± standard deviation) is available for more than one study. For the penicillin G formulations, distinct absorption patterns are observed, depending on which form is administered, the formulation, and the site of injection. The long-acting formulations (procaine- or benzathine–penicillin) are given IM or SC (never IV). The slow absorption from the injection site prolongs the plasma concentration. Injections of penicillin G IM to horses produced prolonged plasma concentrations, even when the sodium salt of penicillin was administered (Uboh et al., 2000). The half-life was longer from the procaine formulation, but at 24 hours, concentrations were similar in horses, regardless of whether the procaine or potassium formulation of penicillin G was administered. Benzathine formulations produce lower, but much longer, plasma concentrations because of insolubility and extremely slow absorption from the injection site (Papich et al., 1994). For all the penicillins, as expected, the maximum plasma concentration will be lower, and the time to reach maximum concentration delayed, from an IM route versus IV route. More rapid absorption occurs from IM injection than SC. Also, there are differences depending on the location in large animals, with an injection in the neck muscle being absorbed faster than an injection in gluteal muscle (Papich et al., 1993). This pattern of absorption has been shown for other penicillins in cattle and horses (Firth, 1986), in which injections in the neck muscle are absorbed more rapidly and completely than injections in the rear leg. For ampicillin and amoxicillin, these can be administered IM or IV as sodium salts, or they may be formulated as the trihydrate form that is more stable in aqueous solutions and may be administered SC or IM to produce a more prolonged absorption phase and a longer duration of activity (Traver and Riviere, 1982). Sodium salts of the other penicillin derivatives are no longer commercially available for IM or IV administration (e.g., ticarcillin sodium, piperacillin sodium, etc.). Penicillin is easily inactivated in the acidic pH of the stomach (a pH of 6–6.5 is optimum for chemical stability), and therefore is not absorbed orally (except the acid-stable penicillin V, which is discussed under the specific formulations below). Because oral absorption of penicillin G is not sufficient to be of therapeutic value in animals this route is not used. Amoxicillin and ampicillin are often administered to small animals. Amoxicillin differs from ampicillin only by the addition of a hydroxyl group (Figure 33.2). This decreases the lipophilicity of amoxicillin, but increases the oral absorption, whereby systemic availability of amoxicillin administered orally is higher than a similar dose of ampicillin (Watson et al., 1986). For example, absorption of ampicillin in dogs and cats has been reported to range from 30 to 40%, and for amoxicillin 64 to 68% (Küng and Wanner, 1994). Ampicillin had wide variation of oral absorption in cats (18% for suspension; 42% for capsule), depending on the dosage form (Mercer et al., 1977). Amoxicillin also has twice the systemic bioavailability of ampicillin when administered orally in pigs and preruminant calves. Other penicillin derivatives may have low oral absorption, which limits their clinical usefulness. Cloxacillin is absorbed poorly in dogs (Watson et al., 1986; Dimitrova et al., 1998) and with its short half-life is unsuitable for therapy in dogs. Figure 33.2 Structure of ampicillin (left) and amoxicillin (right). The effect of feeding on oral absorption of these drugs has been debated, and results have varied, depending on the conditions of the study. The most complete study on this subject was reported by Watson et al. (1986). They found that feeding dogs inhibited oral absorption of amoxicillin, ampicillin, penicillin V, and cloxacillin. The effect was modest for ampicillin and amoxicillin (feeding decreased ampicillin tablets by 38%, but only 20–25% for amoxicillin). Feeding had a large effect for cloxacillin, decreasing oral absorption by 74%. Aminopenicillins are absorbed poorly in horses and ruminants following oral administration. They can be absorbed in preruminant calves, but not ruminating (6 weeks of age) calves (Soback et al., 1987a). Oral absorption of ampicillin in adult horses has been reported to be only 2–3.5% (Ensink et al., 1996; Sarasola and McKellar, 1994). Systemic availability of oral amoxicillin is higher than ampicillin in adult horses but is only 2–10% (Wilson et al., 1988; Ensink et al., 1992, 1996; Baggot, 1988; Sarasola and McKellar, 1994); still too low to be practical for dosing. In foals, oral absorption of amoxicillin has been higher at 36.2–42.7% (Baggot et al., 1988), but this is a seldom-used route of administration. The elimination half-life for IV penicillin is short (0.5 to 1.2 hours). Slow-release formulations are designed to lengthen this half-life. For example, because of slow release from the injection site, procaine penicillin may produce a terminal half-life of 20 hours or more and can maintain concentrations against susceptible bacteria for 24 hours after a single injection. The prolonged terminal half-life is caused by slow absorption (see the Section Absorption), rather than slow elimination, which is referred to as the “flip-flop effect” determining the plasma profile. All penicillins rely on renal elimination (primarily tubular secretion) and reach high drug concentrations in the urine. Penicillins diffuse into extracellular fluid easily unless protein binding is high. Protein binding is low to moderate in most species, ranging from approximately 30 to 60%. Penicillin is a weak acid with a pKa of 2.7, therefore it is mostly ionized in the plasma and the volume of distribution is moderate. For example, volume of distribution (Vd) listed in some studies is 0.2 to 0.3 l/kg. In some studies, and in some species, however, it may be as high as 0.6 to 0.7 l/kg. These values represent a distribution to extracellular fluid, and possibly reaching moderate intracellular concentrations. Sufficient concentrations are attained for susceptible bacteria in kidneys, synovial fluid, liver, lung, skin, and soft tissues (Stover et al., 1981; Brown et al., 1982; Beech et al., 1979). Penicillins do not penetrate the blood–brain barrier to reach concentrations in the central nervous system (CNS) to a large extent, but they have been used to treat infections of the CNS when administered at high doses. Penicillin G, penicillin V, nafcillin, ticarcillin, and the aminopenicillins are metabolized to some extent by hydrolysis of the β-lactam ring. The metabolites are microbiologically inactive. Penicillins and their metabolites are excreted in the urine by tubular secretion. Most of the drug is excreted in the urine within 1 hour of IM injection of sodium or potassium penicillin in aqueous solution. Probenecid competitively inhibits renal tubular secretion of penicillins and can prolong the half-life of penicillin. However, probenecid is rarely used for this purpose in clinical situations. The only natural penicillins still in use are penicillin G and penicillin V. Penicillin V has a phenoxymethyl group that provides more acid stability in the stomach, allowing for oral administration. It has been used orally in people, but it is of limited value in animals. It had low oral absorption and limited spectrum in calves (Soback et al., 1987b). In dogs, oral administration of penicillin V tablets produces maximum plasma concentrations of 3.5–4.8 μg/ml, but the concentrations decline quickly and were above 0.5 μg/ml for only approximately 3 hours (Watson et al., 1987a, 1987b). There are three injectable formulations. Pharmacokinetics of these formulations are provided in Table 33.3 : Additional formulations and route of administration are the formulations of penicillin G administered via intramammary routes to treat bovine mastitis. The doses of penicillin G vary greatly depending on the formulation, the species of animal, and the disease treated. It is best to consult references related to the specific disease being treated. In general, Na+ or K+ penicillin G are administered IM, or IV at doses of 20,000 to 50,000 U/kg every 4 to 6 hours. Procaine penicillin G is administered IM or SC at dosages of 22,000 to 70,000 U/kg, every 12 to 24 hours. Treatment of streptococcal infections may use a lower dose, but for some infections such as those caused by Arcanobacterium (formerly called Actinomyces) doses as high as 100,000 U/kg have been recommended. The United States FDA–approved label dose for cattle is 7,500 U/kg, but the approved withdrawal time for food animals – 10 days – applies only to an approved food animal dose. Since the doses used in food animals are extralabel, extended withdrawal times must be applied. Food animal residues and withdrawal times are discussed further in Chapter 61 of this book. Ampicillin and amoxicillin have been used in the treatment of a variety of diseases in domestic animals. The half-life of all aminopenicillins is short (Table 33.2), requiring frequent administration for some infections, particularly gram-negative pathogens that may have high MIC values. Aminopenicillins are popular because they have a broader spectrum of activity compared to penicillin G, can be administered orally, and are relatively inexpensive and safe. The aminopenicillins differ from penicillin by the addition of an amino group, and amoxicillin has a parahydroxy group that ampicillin lacks. Compared to penicillin G, these compounds have two ionization points (pKa 2.7 and 7.3). Ampicillin is more soluble than amoxicillin, and is also more lipophilic (Log P ampicillin 1.35; Log P amoxicillin 0.87), but amoxicillin is better absorbed by a factor of approximately two in most animals. Compared to penicillin G, the aminopenicillins can penetrate the outer layer of gram-negative bacteria better than penicillin G; therefore, they have a spectrum of activity that includes those listed for penicillin but is extended to include some of the gram-negative bacteria (e.g., susceptible Enterobacteriaceae). However, acquired resistance can be common and this group is still quite susceptible to β-lactamase. To overcome resistance the β-lactamase inhibitors clavulanic acid and sulbactam have been added to amoxicillin (Clavamox) and ampicillin (Unasyn), respectively, to increase the spectrum. These are discussed in section β-Lactamase Inhibitors. The formulations of aminopenicillins used in veterinary medicine (examples of brand names in parentheses) that are available include: The elimination of the aminopenicillins is slightly longer than that reported for sodium salts of penicillin, but this difference does not appear to relate to a difference in clinical efficacy. Common doses are listed in Table 33.4, and listed for breakpoints in Table 33.1. A common formulation used in animals is ampicillin trihydrate (Polyflex), a poorly soluble preparation. After IM injection, the half-life is 6.7 hours in cattle, which is adequate for once-daily administration (Gehring et al., 2005). The IM injection route in horses also prolongs the plasma concentration (Table 33.2). Table 33.4 Common dosages for ampicillin and amoxicillin An important difference between the aminopenicillins and penicillin G is that the aminopenicillins are not inactivated by gastric acid and may be administered orally. There appears to be a saturable transport process for absorption of aminopenicillin in the intestine. Oral absorption of the penicillins in various species and effect of feeding and age is discussed in the Section Absorption. In addition to the formulations listed above, there are ester derivatives of ampicillin, but these are not currently available. The advantage of these esters is that they are stable in the gastrointestinal tract and are absorbed intact, but esterases release the active drug after absorption across the intestinal mucosa. They are absorbed much better than the parent drugs. Examples of these drugs are pivampicillin and bacampicillin (Sarasola and McKellar, 1994; Ensink et al., 1996). Also called the β-lactamase stable penicillins, this group of antibiotics includes the isoxazolylpenicillins (e.g., oxacillin, cloxacillin, and dicloxacillin) and synthetic derivatives of penicillin (e.g., methicillin and nafcillin). These drugs are rarely used clinically in veterinary medicine (except for intramammary forms). These drugs have the disadvantage of poor or inconsistent oral absorption in animals and short half-life. More details on these drugs are provided in earlier editions of this book. They are resistant to the β-lactamase of Staphylococcus
β-Lactam Antibiotics: Penicillins, Cephalosporins, and Related Drugs
Mechanism of Action of β-Lactam Antibiotics
Pharmacokinetic–Pharmacodynamic (PK-PD) Properties
Microbial Resistance to β-Lactam Antibiotics
β-Lactamase Enzymes
Staphylococcal β-Lactamase
Gram-Negative β-Lactamases
Resistance Caused by Reduced Access to Binding Sites
Penicillins
General Pharmacology
Unitage
Structure
Antimicrobial Activity
Susceptibility Testing
MIC interpretive criteria (μg/ml)
Species
Body site
Antimicrobial agent
S
I
R
Comments
Dogs
Skin, soft tissue (E. coli)
Ampicillin
≤ 0.25
0.5
≥ 1.0
Ampicillin may be used to test for amoxicillin. Systemic breakpoint derived from microbiological, PK-PD data. For dogs, the dose of amoxicillin was 22 mg/kg every 12 hours orally.
Dogs
Skin, soft tissue (gram-positive cocci)
Ampicillin
≤ 0.25
–
≥ 0.5
Ampicillin is used to test for susceptibility to amoxicillin and hetacillin. Systemic breakpoint derived from microbiological, PK-PD data. For dogs, the dose of amoxicillin was 22 mg/kg every 12 hours orally.
Dog
UTI
Ampicillin
≤8
–
–
A breakpoint of ≤8 can be used for uncomplicated UTI to account for high concentration in urine.
Dogs
Skin, soft tissue
Amoxicillin–clavulanate
≤0.25/ 0.12
0.5/0.25
≥1/0.5
Amoxicillin–clavulanate breakpoints were determined from an examination of MIC distribution of isolates, efficacy data, and PK-PD analysis of amoxicillin in dogs. The dosage regimen used for PK-PD analysis of amoxicillin was 11 mg/kg administered every 12 hours orally.
Dogs
UTI
Amoxicillin–clavulanate
≤8/4
A breakpoint of ≤8/4 can be used for uncomplicated UTI to account for high concentration in urine.
Dogs
Skin, soft tissue, UTI
Piperacillin–tazobactam
≤8/4
≤16/4
≤32/4
Breakpoint was derived from an examination of MIC distribution data, and PK-PD analysis of piperacillin in dogs at a dosage of 350 mg/kg every 6 hours, intravenously, or 3.2 mg/kg/hour constant rate IV infusion.
Cats
Skin, soft tissue, UTI
Amoxicillin–clavulanate
≤0.25/ 0.12
0.5/0.25
≥1/0.5
Amoxicillin–clavulanic acid breakpoints were determined from an examination of MIC distribution of isolates, efficacy data, and PK-PD analysis of amoxicillin in cats at a dosage of 12.5 mg/kg (amoxicillin) administered every 12 hours orally.
Horse
Respiratory, soft tissue
Penicillin G
≤ 0.5
1
≥ 2
Breakpoints derived from microbiological, PK data (using accepted clinical, but extralabel doses), and PD data. The dose of procaine penicillin G modeled was 22000 U/k, IM, every 24 hours.
Horse
Respiratory Disease
Ampicillin
≤ 0.25
–
–
For strains yielding results suggestive of a “nonsusceptible” category, organism identification and antimicrobial susceptibility test results should be confirmed.
Swine
Lung (SRD)
Ampicillin
≤ 0.5
1
≥ 2
Breakpoints derived from microbiological data using ampicillin, PK data from a dose of 15 mg/kg IM of amoxicillin once daily, and PD data.
Swine
Lung (SRD)
Penicillin G
≤ 0.25
0.5
≥ 1
Breakpoints derived from microbiological, pharmacokinetic data (using accepted clinical, but extralabel doses), and pharmacodynamic data. The dose of procaine penicillin G modeled was at a dose of 33,000 U/kg, IM by needle in the neck, every 24 hours.
Cattle
Lung (BRD)
Penicillin G
≤ 0.25
0.5
≥ 1.0
Breakpoints derived from microbiological, PK data (using accepted clinical, but extralabel doses), and PD data. The dose of procaine penicillin G modeled was 22000 U/k, IM, every 24 hours.
Cattle
Mastitis
Penicillin–novobiocin
≤ 1/2
2/4
≥4/ 8
Mastitis use only.
Pharmacokinetics
Species
Dose (mg/kg)
T ½ (hour)
Vd (l/kg)
CL (ml/kg/hour)
Cmax (μg/ml)
Tmax (hour)
F (%)
Reference
Ampicillin trihydrate IM administration
Calves
11
2.62
Martinez et al., 2001
Pigs
6.6
3.25
Martinez et al., 2001
Horse
11
1.48
1
Beech et al., 1979
Horse
22
2.9
6
Beech et al., 1979
Horse
20
2.49
6
Brown et al., 1982
Cattle
17
6.66
4.49
467.8
Gehring et al., 2005
Ampicillin sodium IV
Horses
0.62
0.18
210
6.7–9.7
Sarasola and McKellar, 1993
Horses
10
0.725
0.303
268
59.9
Sarasola and McKellar, 1992
Horses
1.55
Durr, 1976
Horses
1.41
0.17
Bowman et al., 1986
Horses
0.75
0.21
Horspool et al., 1992
Horses
15
1.72
0.705
285
Ensink et al., 1992
Horses
10
0.7
0.2628
365.4
Sarasola and McKellar, 1995
Cats
1.22
Mercer et al., 1977
Pigs
0.55
Galtier and Charpenteau, 1979
Dogs
15
1.35
0.679
387
ten Voorde et al., 1990
Sheep
10
0.78
0.156
372.6
Oukessou and Toutain, 1992
Ampicillin Sodium IM
Cows
10
2.3
6.18
1.5
Nelis et al., 1992
Horses
15
2.3
0.71
209.8
31.1
0.32
Van Den Hoven et al., 2003
Horses
11
10
0.5
Beech et al., 1979
Horses
22
2
12.88
0.5
Traver and Riviere, 1982
Dogs
15
5.2–5.5
0.92–1.03
ten Voorde et al., 1990
Ampicillin Oral
Dogs
14.5
0.9
4.6
Nelis et al., 1992
Dogs
10
0.96
3.9
1.6
Watson et al., 1986
Foal
20
5.0
1
Brown et al., 1984
Cat
42
Mercer et al., 1977
Horse
15
0.84
0.69
2
Ensink et al., 1996
Amoxicillin
Foals (30 days) IM
22
0.991
0.986
691
23.21
Carter et al., 1986
Foals (6–7 days) IV
20
0.74
0.369
343.2
Baggot et al., 1988
Foals (6–7days) oral
20
1.09
6.23
2
36.2
Baggot et al., 1988
Horses IV
10
0.657
0.325
340.8
Wilson et al., 1988
Horses IM
3.9–11.9
Evans et al., 1971
Horses oral
20
0.85
11.05
0.3
10.4
Wilson et al., 1988
Horses oral
20
0.75
2.03
5
Ensink et al., 1992
Horses IV
10
1.43
0.556
273
Ensink et al., 1992
Dogs IV
20
1.3
0.312
204
Küng and Wanner, 1994
Dogs IV
15
1.18
0.449
270
ten Voorde et al., 1990
Dogs IM
15
6.98–9.02
7.64–8.13
1.61–1.89
ten Voorde et al., 1990
Pigs IV
8.6
1.8
0.55, 0.63
370, 520
Agersø and Friis, 1998
Pigs IM
14.7
15.5
5.1
2
83
Agersø and Friis, 1998
Sheep IV
10
0.77
0.22
606
Craigmill et al., 1992
Sheep IV
20
1.43
0.18
90
Carceles et al., 1995
Goats IV
10
1.15
0.47
684.6
Craigmill et al., 1992
Goats IV
20
1.13
0.18
110
Carceles et al., 1995
Dogs oral
1.06
0.284
182
Marier et al., 2001
Dogs oral
16.9
1.52
0.71
460
11.4
1.38
Vree et al., 2003
Dogs oral
21
1.5
18–21
1.4–2
64–77
Küng and Wanner, 1994
Dogs oral
10
1.4
8.1
1.6
Watson et al., 1986
Pigs oral
10
9, 9.9
2.25
0.8, 1.6
1.9, 3.6
28, 33
Agersø et al., 1998
Pigs oral
20
4.2
7.5
1.5
Jensen et al., 2004
Species
Form
Dose (Units/kg)
Route/Site
Cmax (μg/ml)
Tmax (hours)
T½ (hours)
Reference
Calves (6–9 months)
Potassium
10,000
IM/Neck
4.71 ± 3.86
1 to 1.5
–
Bengtsson et al., 1989
Calves (6–9 months)
Procaine
30,000
IM/Neck
1.55 ± 0.33
1.5 to 6
–
Bengtsson et al., 1989
Cattle
Procaine
66,000
IM/Neck
4.24 ± 1.08
6.00 ± 0.00
8.9
Papich et al., 1993
Cattle
Procaine
66,000
SC/Neck
1.85 ± 0.27
5.33 ± 0.67
17
Papich et al., 1993
Cattle After 5-day administration
Procaine
24,000
IM/Gluteal
0.99 ± 0.04
5.33 ± 0.67
17
Papich et al., 1993
Cattle
Procaine
66,000
IM/Gluteal
2.63 ± 0.27
6.00 ± 0.00
17
Papich et al., 1993
Cattle
Procaine
9.37 ± 3.4
Craigmill et al., 2004a
Horses
Sodium
10,000
IV/Jugular
Love et al., 1983
Horses
20,000
IV/Jugular
Horses
40,000
IV/Jugular
Horses
Procaine
10,000
IM/Gluteal
Sullins et al. 1984
Horses
20,000
IM/Gluteal
Horses
40,000
IM/Gluteal
Horses
Procaine
22,000
IM/Gluteal
1.42 ± 0.22
3
Stover et al., 1981
Horses
Procaine
22,000
IM/neck
1.8
3.5
24.7
Uboh et al., 2000
Horses
Potassium
22,000
IM/neck
5.8
1
12.9
Uboh et al., 2000
Horses
Procaine
20,000
IM
1.57 ± 0.44
4.77 ±0.26
16.4 ± 8.0
Mean of 3 studiesb
Foals (0–7 day)
Procaine
22,000
IM Semimembranous
2.17 ± 0.27
2
Brown et al., 1984
Pigs
Procaine
15,000
IM neck
1.47
1.20
7.78
Papich (unpublished)
Pigs
Procaine
66,000
IM neck
5.40
1.0
14.13
Papich (unpublished)
Absorption
Elimination
Distribution
Metabolism
Summary of Penicillins and Derivatives
Natural Penicillins
Penicillin formulations:
Aminopenicillins
Aminopenicillin formulations:
Drug
Dose and species
Amoxicillin oral
Dogs, cats 6.6–20 mg/kg q 8–12 h
Ampicillin trihydrate injection
Cattle 6.6–22 mg/kg q 8, 12, or 24 h (24 h most common); the approved label dose in cattle is 4.4–11 mg/kg IM, q 24 h Dogs, cats: 10–20 mg/kg, q 12–24 h, IM, SC
Ampicillin sodium
Horses IV 10–20 mg/kg q 6–8 h
Ampicillin sodium
Horses IM 10–22 mg/kg q 12 h
Ampicillin sodium
Dogs, cats: 10–20 mg/kg, q 8 h, IV, IM, or SC
Antistaphylococcal Penicillins
Stay updated, free articles. Join our Telegram channel
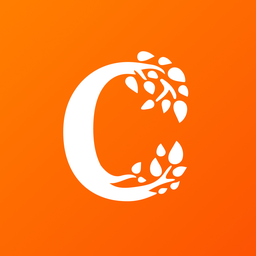
Full access? Get Clinical Tree
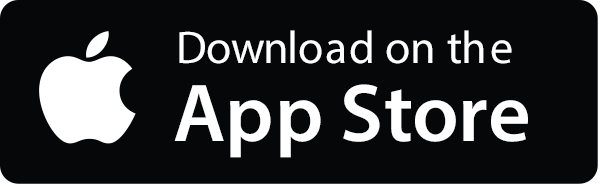
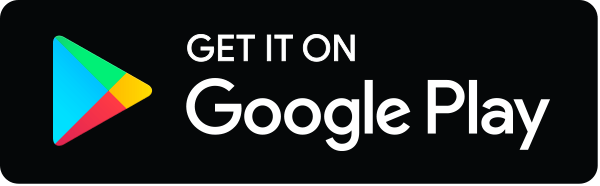