Fig. 1.
A schematic drawing of the telencephalon of ray-finned fish illustrating the subdivisions of the telencephalon (left) in a coronal section. On the right is a presentation of how the fish anatomical structures are thought to correspond to mammalian structures. Dd dorsal region of area dorsalis telencephali, Dm medial region of area dorsalis telencephali, Dl lateral region of area dorsalis telencephali, Dc central region of area dorsalis telenchphali.
The brain structure in zebrafish that is laminar is the optic tectum, which corresponds to the superior colliculus in the midbrain, mesencephalon, of mammals. In both mammals (11) and zebrafish (12), this structure integrates visual information with motor output, and in zebrafish this area drives prey capture behavior (13).
The brainstem nuclei are in a critical position to regulate lower motor neurons and mediate modulatory inputs from telencephalic and cerebellar systems in mammals. The neurons and neuronal networks in the brainstem and spinal cord have been the focus of movement studies in zebrafish; and in the following chapters, we will review the work further.
The role of the systems that modulate motor responses, such as basal ganglia and cerebellum in zebrafish are largely unknown. Based on a tracing study and gene expression data, basal ganglia, including the striatum, are suggested to be located in the ventral telencephalon (7, 14), whereas toxicity studies, neuronal connectivity, and tracing studies suggest substantia nigra to be located in the posterior tuberculum of zebrafish (15–17). In mammals, the thalamic complex is the largest structure of the diencephalon and consists of the prethalamus, zona limitans intrathalamica, and thalamus. Comparative analyses of mouse, chick, and zebrafish revealed that the development of the thalamus is evolutionarily conserved and that sonic hedgehog plays a crucial role in the formation and differentiation of thalamic nuclei (reviewed in (18)). A double labeling study in zebrafish showed that the main dopaminergic ascending innervation from the posterior tuberculum to the ventral telencephalon was from the periventricular nucleus of the posterior tuberculum (14). The cerebellum of teleosts differs from the mammalian cerebellum, as in teleosts eurydendroid cells interact with the Purkinje cells, whereas in mammals the axon of the Purkinje cells interact with the deep cerebellar nuclei (19). To study the role of the cerebellum in the modulation of higher brain functions and behavior, the anatomy of the cerebellum in developing and adult zebrafish as well as mutations that affect the development of the cerebellum was recently compiled (20).
Genetic approaches combined with the transparency and the small size of the zebrafish larvae are features that render it a good model organism for studying the neuronal circuits underlying movement. Targeting and stimulating and/or ablating single neurons in an intact vertebrate organism and assessing the role of specific neurons in different types of behaviors is possible in zebrafish larvae (21). Calcium imaging can be used to assess which neurons mediate specific behaviors. An increase in calcium levels, which can be detected by several Ca2+ indicators, reflects the activity of a neuron in response to specific stimuli (22, 23). Optogenetics is another approach that allows activation of neurons within millisecond range providing a tool to study the role of targeted neurons in specific behaviors (24, 25) (see also Chap. 6 this volume). Quantification of the behavioral responses can be recorded by live imaging of the zebrafish and correlated to neuron activation measured with optical or electrophysiological methods. High-throughput drug screening combined with a behavioral readout (26) is another appealing approach for studying behavior and pharmacological correlates in this vertebrate model organism.
2.1 Neurotransmitter Systems in Zebrafish Brain
The neurotransmitter systems relevant for motor control and movement disorders are very similar in zebrafish and mammals. Thus, the major biogenic amine systems are well conserved and the CNS domains, which harbor cell body groups, are similar, with a few exceptions (27). Contrary to invertebrates such as D. melanogaster and C. elegans, where receptor mechanisms are very different from mammals despite the similar nature of many neurotransmitters, the zebrafish neurotransmitter receptors are in general very alike those of mammals. Genome duplication has resulted in expansions of some receptor families, and the total number of G-protein-coupled receptors in zebrafish is larger than that of mammals (for a detailed description, see (27)). For example, the three α2 receptor subtypes (α2a–c) are similar to those of mammals, but there is a fourth duplicated receptor that is not found in mammals (α2da and αdb) (28, 29). The potentially duplicated α2a–c receptors have been lost during zebrafish evolution. Genes corresponding to the three histamine receptors (hrh1–3), known to be important in mammalian brain functions (30), are found in zebrafish and expressed in the brain (31), and their ligands have significant effects on zebrafish locomotor activity.
The rate-limiting enzyme tyrosine hydroxylase (TH) is duplicated in zebrafish (32), and this phenomenon has not been noted in most papers that deal with the zebrafish dopaminergic system. Thus, most studies which deal with neurotoxicological or genetic manipulation of the dopamine system, only TH1 has been examined, since also available antibodies detect this form efficiently (33). There is now evidence that TH2 expressing neurons, in addition to those which express TH1, are affected by translation inhibition of PINK1, a mitochondrial kinase important in autosomal hereditary Parkinson’s disease (34). The expression patterns of TH1 and TH2 are complementary. TH2 is expressed in four major bilateral clusters in the preoptic region and hypothalamus (33), whereas a total of 17 cell groups in the whole brain express TH1 (17). Recently, we have developed an antibody against TH2, which shows that the TH2-expressing cells produce TH protein and the cells display a distinct morphology (Semenova et al., in preparation).
Whereas mammals have two distinct monoamine oxidase genes, the zebrafish genome encodes for one enzyme which appears functionally as a hybrid of the two mammalian forms (35), with eight of the 12 residues of the active site identical with human MAO A and MAO B, two with human MAO A and two appear unique for zebrafish (35). MAO mRNA is expressed early (at 24 hpf) in developing zebrafish, and enzyme activity is measurable at 42 hpf (36). Inhibition of zebrafish MAO with deprenyl strongly elevates brain 5-HT content and decreases swimming activity sharply in a dose-dependent manner (36). This effect is due to release and uptake of serotonin in non-serotonergic neurons in basal hypothalamus (36). It is important to take this into account in, e.g., toxicology experiments with 1-methyl-4-phenyl-1,2,3,6-tetrahydropyridine (MPTP), which require conversion to 1-methyl-4-phenylpyridinium (MPP+) by MAO to induce a decline in motility in both larval (17) and adult (37) zebrafish.
2.2 Brainstem and Spinal Cord Systems in Zebrafish
The brainstem of zebrafish larvae contains approximately 150 reticulospinal neurons that project to spinal cord to regulate execution of an array of different behaviors and movement patterns. Of these reticulospinal neurons, the Mauthner neuron, discovered in 1859 by Ludwig Mauthner, is the most famous and is thought to be the sole driver of the escape response, which also is called the startle response. The startle response of freely moving larval zebrafish has been characterized and elegantly demonstrated to include a short latency and a long latency startle response (21). Both types of startle response are short lasting and completed in less than 1 s after stimulation. Laser ablation of the Mauthner neuron abolished the short latency startle response, whereas the long latency startle responses were unaltered, indicating that only the short latency startle response is mediated by the Mauthner neuron. Other neurons of the brainstem such as MID2cm (Mauthner neuron homologue, middle dorsal 2 contralateral medial longitudinal fasciculus interneuron in rhombomere 5) and MID3cm (Mauthner neuron homologue, middle dorsal 3 contralateral medial longitudinal fasciculus interneuron in rhombomere 6) cooperate with the Mauthner neuron to provide the escape response with a direction (22). Stimulation of the head versus the tail elicits activity in a specific but different manner in Mauthner neurons and MID2cm and/or MID3cm neurons. In the case of a head stimulus both Mauthner neuron and MID2cm or MID3 neuron respond, whereas when the tail is stimulated only the Mauthner neurons are activated. Orger et al. (23) studied the role of specific reticulospinal neurons in turning behaviors and slow swimming, and found forward-preferring neurons among the neurons of the medial longitudinal fasciculus, nucMLF. In the same study, neurons that execute right or left movements were also identified. A schematic drawing of the anatomical position of the described neurons is presented in Fig. 2, and also their connections via commisural interneurons to lower motor neurons in the spinal cord are illustrated.
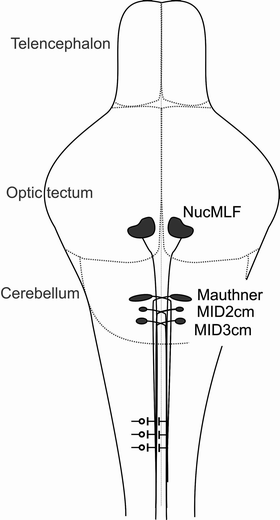
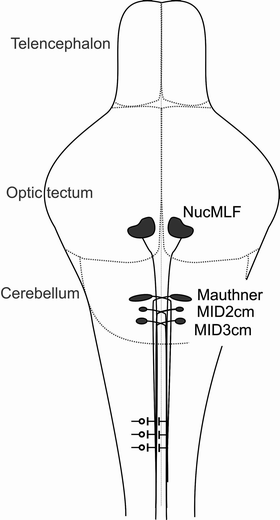
Fig. 2.
A schematic picture of some reticulospinal neurons in zebrafish brainstem. NucMLF, Mauthner neuron, MiD2cm, and MiD3cm are illustrated as examples of reticulospinal upper motor neurons, because the behaviors driven by these neurons have been identified (21–23). Their location is correlated to telencephalon, optic tectum, and cerebellum. In the spinal cord commissural interneurons and connections to lower motor neurons are illustrated.
2.3 Movement Analysis of Zebrafish Larvae
Zebrafish larvae show spontaneous movement as early as 17 hpf (2). At 3 dpf, the fish show bursts of activity, but the continuous beat-and-glide swim pattern is observed at the earliest at 4 dpf. As the fish grows older the time spent swimming increases. By 5 dpf larvae have almost consumed the nutrients in their yolk sack and need to start hunting for food in order to survive. At this stage different behaviors can be observed, such as prey capture (41) and sleep (42, 43).
In order to link neurons to circuits and behaviors, behaviors can be dissected into smaller units or movement patterns. Kinematic analysis, which includes dissection of specific behaviors into different movement patterns, provides a detailed description of the repertoire of movements that the zebrafish combines to produce specific behaviors. For dissecting the kinematics, it is essential to observe different behaviors or movement patterns of zebrafish using high frequency imaging (starting at 500–1,000 frames/s). Behaviors that need to be assessed with high frequency imaging are very rapid, lasting only for milliseconds, e.g., startle response and prey capture. For other movement patterns and behaviors such as place preference, sleep, or motility assays, which can be followed for long periods (from seconds to days), live imaging with standard video tracking frequency (starting at five samples/s or less) is adequate.
Several studies have addressed the movement pattern repertoire of zebrafish larvae and classified the movement patterns in different manner (41, 44, 45). Zebrafish exhibits an escape response consisting of a C-start turn (a Mauthner cell-driven activation of motor neurons on the contralateral side of the spinal cord enabling the fish to turn and swim away from the stimuli) in response to acoustic/vibrational stimuli (21) and O-bends (a Mauthner cell-independent movement that is slower than the C-start turn) in response to dark flashes (46). For navigational purposes, adjustment moves, turns, and scoots (45), and slow-swim-like patterns with the pectoral fins can be detected (41). These movement patterns can be detected with high frequency imaging, which requires specific cameras that can acquire images at a high speed (500–1,000 frames/s) and lenses with resolution to image larvae. Strong light, either the infrared or the visual light wavelength range, is also needed as the contrast between the larvae and background needs to be high enough to distinguish the larvae. As the behaviors that are detected are very rapid, it is an advantage if the stimuli and recording of the behavior can be synchronized. Several individuals can be recorded in the same group, and when the images have been captured, the different movement patterns can be identified from the images.
Above, we already reviewed how the escape response is divided into two different startle responses. Another robust response of the larval zebrafish is the prey capture behavior, which consists of combining the movement patterns described above. Paramecia are often used as live food for rearing zebrafish, and the prey capture behavior of zebrafish larvae has been studied by high frequency imaging when exposing paramecia to the fish (41). As a consequence, the larvae orient themselves in response to the position of the paramecia by small discrete movements, including routine-like turns to align the body axis of the larvae with the paramecia and slow swim-like patterns with the fins to slowly move closer to the prey before engulfing it (41). Prey capture has been shown to be mediated by two specific neurons as bilateral ablation of two neurons, MeLc and MeLr, in the medial longitudinal fasciculus (nucMLF) completely abolished the prey capture without affecting other behaviors (47). Recently, filtering of small visual cues in the optic tectum was demonstrated to be essential to enable prey capture in zebrafish larvae (13).
2.4 Locomotor Analysis of Larval and Adult Zebrafish
Motility assays are the most common behavioral assays used for both larval and adult zebrafish. We and other groups have established methods for detecting the movement and motor functions of both adult (37, 48, 49) and larval zebrafish (17, 26, 31, 36). We have used a computerized video tracking system successfully for almost a decade. A number of modifications have been done to the basic setup in order to observe different aspects of zebrafish behavior. Automated video tracking that converts the live image to graphical representations allows quantification of the movement of fish. In this manner, the behavior of larval and adult zebrafish can be monitored from seconds to days. If the tracking lasts for several days, it is of utmost importance that the conditions, noise, light, humidity, and temperature of the observation room is held at constant and appropriate levels. The basic setup is robust and relatively simple, utilizing both wavelengths from the visual range and/or infrared light for detection of the freely moving pigmented zebrafish. Furthermore, this system offers high throughput, because as many as 96 larvae can be observed simultaneously in a single system.
We have focused on the locomotor functions of zebrafish, such as total distance moved, velocity and frequency of movement. We have also observed the circular movement of zebrafish, such as turn angle, angular velocity, and meander (31, 36, 37, 48). Furthermore, we have assessed behaviors such as dark flash response in larval zebrafish (Sundvik et al., in preparation) and place preference in both adult (37, 48) and larval zebrafish (unpublished observation).
The locomotor behavioral method with its several modifications, has limitations that have to be taken into account, but can easily be mastered. In the following, we deal with the major variables. To start with, it is important to take into account how many individuals are necessary to be included in the experiment, and if both the control and treatment group should be monitored simultaneously or if they can be analyzed subsequently. If a large number of individuals per group is essential, this reduces the number of parameters that can be assessed, as the well size is reduced. If place preference is a priority parameter, it is important to have arenas spacious enough for the fish to be able to show a preference. Usually, for larval zebrafish, we used standard well plates of different size, ranging from 6- to 96-well plates, which result in arena diameter of 36–7 mm, respectively. Most often, we have used the 48-well plates, with arena diameter of 13 mm, as the different parameters of movement and different behaviors can still be distinguished. This format also offers high throughput as the behavior of 48 larvae can be assessed simultaneously. The standard well plates have one major drawback, as they are made of transparent instead of white plastic, the inner surface of the wells need to be modified in order to inhibit formation of mirror images of the larvae on the walls of the wells. Therefore, we have also made arenas of white plastic (this offers better contrast between the larvae and background and does not produce mirror images of the larvae) and have a larger diameter (40 mm) where the place preference behavior of zebrafish larvae is easily monitored. When assessing movement and place preference in adult zebrafish, the same basic principles as for larvae apply. It is important to have spacious enough arenas, the animals should be easily distinguishable from the background, and the method should offer options for tracking the behaviors of several individuals simultaneously. As the adult zebrafish tend to jump, it is important that the walls of the arenas are high enough (at least 8 cm over water level) so that the fish cannot move from one arena to the other. The last aspect is that the tracking systems that utilize only one camera create a two-dimensional representation of the movement pattern of the fish. Therefore, the depth of the water column in which the fish is swimming should be as small as possible when horizontal movement is tracked. Recently, some groups have reported that the anxiolytic effect of drugs can be assessed by observing whether the fish spend time in the upper compartment of the water column or at the bottom of the tank (50). Taken together, our approach for assessing behavior offers a robust and crude measurement of motor functions in developing and adult zebrafish.
Apart from studying the locomotor activity, it is feasible both in larval and adult zebrafish to assess the response the fish show to changes in the environment. This can either be studied by moving white and black stripes unidirectionally under the arena where freely moving fish are held, to analyze optomotor response, or by fixing the zebrafish with, e.g., agar and monitor eye movements (optokinetic response). These two assays have successfully been used to screen for visual system defect mutants (51, 52).
2.5 Zebrafish Mutants with Movement Disorders
Fifteen years ago, the large-scale screen in Tübingen identified 166 zebrafish mutants with abnormal movement (44). The identified mutants that were identified by an abnormal touch response fell into 14 phenotypical groups affecting 48 genes in total, of which 18 genes affected somatic muscle development and the remaining 30 genes were suggested to affect neuronal development. The latter group comprised mutants with reduced motility, circling behavior, and motor circuit deficits. A circling behavior mutant, twitch twice, was identified in this screen as a mutant that only showed right or left turns, rather than alternating turning behavior to both sides of the trunk as wild-type zebrafish to accomplish straight-forward locomotion. The twitch twice mutant carries a mutation in the roundabout receptor family gene, robo3 (53). This class of genes is important for axonal guidance, and in the case of the twitch twice mutant, the axons of the Mauthner neuron did not cross the midline as in normal larvae (53). As a consequence, the Mauthner neuron axons are either on left or right side of the trunk and thereby the larva exhibits a unidirectional behavior. A few years later in another mutagenesis screen, the touchtone mutant was identified. This mutant showed reduced motility and pigmentation defects, and the mutation was shown to be located in the transient receptor potential melastatin 7 (trpm7) gene (54). TRPM7 is an ion channel permeable for Mg2+ and Ca2+ and widely expressed in murine cells. Interestingly, loss of trpm7 has been correlated to parkinsonian dementia (55). In zebrafish trpm7 mutants the pigmentation deficit was induced by necrosis of melanophores. The cell death could be inhibited by inhibition of melanin synthesis. As melanin and catecholamines share the same metabolic pathway, this finding gives new insight about the mechanisms underlying cell death of pigmented neurons and can offer a new approach for protecting pigmented neurons from cell death.
3 Zebrafish Models of Parkinson’s Disease
3.1 Neurotoxins to Model PD and Other Neural Diseases
A Parkinson’s disease-like condition can be induced by MPTP in humans and some experimental animals including monkeys and mice. It has been used in several species (56–58), and it gives more consistent results than other current toxin models (59). In astrocytes or neurons, MPTP is converted to the toxic metabolite MPP+ by monoamine oxidase, and this metabolite enters dopaminergic neurons by dopamine transporter to cause death of cells in substantia nigra in mammals by inhibition of complex 1 of the mitochondrial respiratory chain. In adult zebrafish, MPTP causes a delayed, partly transient decline in dopamine and noradrenaline levels, and a locomotor defect characterized by slower movement and abnormal swim tracks (37). 6-Hydroxydopamine, which acts as a false transmitter in dopaminergic neurons, causes grossly similar alterations in dopamine levels and locomotor behavior (37). These motor effects of can be analyzed in detail with a video tracking system, which takes 5–25 frames/s (37, 60), but the fast fine movements and startle responses of the fish are beyond the sensitivity of this method. The histaminergic system is another diffuse projection system also in zebrafish like in other vertebrates (61 62), which has cell bodies in the posterior basal hypothalamus of the fish (63), and widespread projections throughout the brain, most extensively to the dorsal telencephalon and optic tectum (15 39). An irreversible inhibitor of the synthesizing enzyme histidine decarboxylase, alpha-fluoromethylhistidine, induces a significant decline in brain histamine in adult zebrafish and an alteration in swim tracks in a spherical aquarium, without affecting swimming speed (48). To detect this behavioral alteration, the swimming arena needs to be large enough to be divided into zones.
MPTP has been reported to cause cell death in larval zebrafish of the pretectal dopaminergic cell population at 5 dpf (64), the putative posterior tuberal nucleus at 2 dpf (65), and the diencephalic dopaminergic neurons at 5 dpf (66). These studies do not define the diencephalic dopaminergic cell populations anatomically, which renders it challenging to correlate the findings to motor behavioral abnormalities and compare the results of different studies. In one study, specific identified cell populations were affected, and exact decreases in distinct diencephalic clusters were reported following MPTP or MPP+ administration (17). Lam et al. (65) describe cell loss at 2–3 dpf in their study. At this early stage, the MAO activity is not yet detectable in all brain areas (36), so that the mechanism of toxicity may not require conversion to MPP+ and is thus different from that of later larval stages. This is supported by a study by Thirumalai and Cline (67), which found that dopamine inhibits initiation of fictive swimming at 3 dpf, and the effect was antagonized by MPTP and D2 receptor antagonist. Several dopaminergic cell populations are not yet fully developed at 2–3 dpf, and the actions of dopamine are different at different early stages of zebrafish. One of the slowly developing cell groups is the cluster containing the putative population corresponding to mammalian mesencephalic substantia nigra neurons (15, 68), referred to in a detailed anatomical study as population 5,6,11 (17). Now that the th2 expressing cells are known to reside in this area as well (33, 69), the cells targeted by MPTP could also belong to this group. There are currently no published reports on the effect of MPTP on th2 cells, but the diencephalic th2 neurons seem to be sensitive to MPTP (Chen et al., in preparation).
Decreases in dopamine, noradrenaline, and serotonin levels in the larval zebrafish after MPTP exposure (17) suggest that both catecholaminergic and serotonergic systems are affected, which agrees with findings in rodents after MPTP exposure (70, 71) and human PD patients (70, 72). MPP+ administration in zebrafish larvae also affected one cell population in the posterior tuberculum (17). In line with the immunohistochemical results, MPP+ did not affect serotonin levels significantly, while it decreased dopamine and noradrenaline levels. Actual cell death in the zebrafish brain following MPTP has not been observed consistently in different studies. Instead, the loss of TH-ir was found to recover rapidly and completely in some studies (17, 37). The reported declines in dopaminergic neurons are based on the smaller size of th1 mRNA expressing cell clusters without counting the cells (64), reduction of in situ hybridization signal for dopamine transporter mRNA at 48 and 72 hpf (65), reduction of fluorescence intensity in transgenic fish expressing EGFP under the vesicular monoamine transporter 2 promoter (73), or counting th1 mRNA expressing cells in the diencephalon of fish larvae at 5 dpf. In the last study, the total number of cells in control fish diencephalon at 5 dpf was under 40, whereas it in another study, carried out with a TH antibody and three-dimensional imaging, was much larger at 7 dpf (17). This suggests that the significant differences may in part depend on methodological issues. Long-term studies are needed to establish if developmental exposure leads to permanent changes in cell numbers.
3.2 Genetic PD Models
The etiology and pathogenesis of Parkinson’s disease are still not well understood despite extensive studies on the mechanisms underlying neuronal degeneration of dopaminergic and other, e.g., serotonergic neurons in the disease. Several of the genes involved in the rare genetic forms of PD have been cloned in zebrafish, and their translation has been inactivated using morpholino oligonucleotides (MOs). Four genes, Park2 (Parkin), Park6 (Pink1), Park7 (DJ-1), and Park8 (LRRK2) have been studied in detail by inactivating their translation (Table 1).
Table 1
Zebrafish studies using morpholino oligonucleotides for translation inhibition of Parkinson’s disease related genes
Gene | Morpholino type | Cells affected | Phenotype | Reference |
---|---|---|---|---|
Parkin (Park2) | spMO | Diencephalic DA cells (th1) | Reduced complex I act Increase sensitivity to MPP+ Normal swimming beh Electron dens mat. in t-tubules | (82) |
PINK1 (Park6) | trMO | Diencephalic DA cells (th1) | Small eyes, curved tails Enlarged brain ventricles Elevated GSK3β activity Early larval death | (77) |
PINK1 (Park6) | spMO < div class='tao-gold-member'>
Only gold members can continue reading. Log In or Register a > to continue
![]() Stay updated, free articles. Join our Telegram channel![]() Full access? Get Clinical Tree![]() ![]() ![]() |