5 James R. Theoret and Bruce A. McClane The versatile pathogenicity of Clostridium perfringens largely derives from the ability of this bacterium to produce a ~17 toxin armory. Four of those toxins – alpha, beta, epsilon, and iota toxins – are termed “major” toxins due to their use in a toxin-typing classification system (Table 5.1) that assigns C. perfringens isolates to one of five types (A–E). Although not used for this classification scheme, several of the remaining ~13 toxins are nonetheless important for the virulence of C. perfringens. This chapter will briefly review the biochemistry, action, and genetics of the most important C. perfringens toxins that have proven or suspected disease involvement. Table 5.1 Toxin typing of Clostridium perfringens C. perfringens alpha toxin (CPA) is a 42.5 kDa single polypeptide with two domains. The ~250-amino-acid N-terminal domain is responsible for catalytic activity (described below), although it is not immunoprotective. The ~120-amino-acid C-terminal domain mediates host cell-binding activity in the presence of calcium and is immunoprotective. Recent studies reported that alpha toxin can bind to ganglioside GM1 on host cells. CPA was the first bacterial toxin shown to have enzymatic properties. This toxin is a zinc metallophospholipase exhibiting both phospholipase C (lecithinase) and sphingomyelinase activities. CPA is lethal, with an LD50 in mice of 3 μg/kg (Table 5.2). At high concentrations, the toxin can substantially damage plasma membranes to directly lyse host cells. However, at sublytic concentrations, CPA induces more limited cleavage of phosphotidylcholine and sphingomylelin, creating diacylglycerol and ceramide (Figure 5.1). Those molecules then activate several host signal transduction pathways which, via potent second messengers, induce hemolysis and a myriad of other effects, some of which are described below. An interesting recent study suggests that CPA may also be endocytosed, where it might act intracellularly. Table 5.2 Summary of major C. perfringens toxin properties 1LD50 values determined from Gill (1982) and Uzal et al. (2010). 2The reported TpeL LD50 was determined using an unusually weak TpeL variant; the actual LD50 for this toxin is likely to be much lower.ND: not determined Figure 5.1 Activities of various Clostridium perfringens toxins on host cells. See text for a detailed explanation of their specific mechanism of action. Based on results from molecular Koch’s postulate analyses, CPA is considered to be the most important toxin when C. perfringens causes gas gangrene (clostridial myonecrosis). During histotoxic disease, CPA impairs leukocyte migration and increases the adhesion of leukocytes to form intravascular aggregates that impair blood flow and thereby promote necrosis. Since this necrotic tissue environment is anaerobic, it favors C. perfringens growth. Similar analyses using alpha toxin null mutants of NetB-positive type A or type C natural intestinal disease strains suggest CPA is much less important when C. perfringens causes disease originating in the intestines. CPA is encoded by the cpa (also referred to as plc) gene, which is chromosomally located. This toxin is produced by almost all C. perfringens strains, regardless of their type. Production of CPA is regulated by the VirS/VirR two-component regulatory system (TCRS). The cpa (plc) gene does not have VirR boxes upstream of its promoter, so this VirS/VirR regulation is indirect, involving a regulatory RNA named VR-RNA. Recently, expression of CPA was also shown to involve regulation by the Agr-like quorum-sensing (QS) system. C. perfringens beta toxin (CPB) is expressed as a 336-amino-acid prototoxin, containing a 27-amino-acid leader sequence that is subsequently removed during secretion from the cell to generate a mature ~35 kDa polypeptide. The structure of CPB has not been resolved, but based upon its partial sequence homology with other toxins, CPB is considered a member of the β-pore-forming toxin (β-PFT) family. Like all β-PFTs, CPB is predicted to contain three functional domains: binding, oligomerization, and membrane insertion/pore formation (see below). CPB induces cell death by creating unregulated ion channels, with a pore size of approximately 12 Å, in host cell membranes. These channels permit rapid K+ efflux, and Na+, Ca2+, and Cl– influx, resulting in cellular swelling and lysis (Figure 5.1). Pore formation begins with the binding of CPB to host cells using a still unidentified receptor. Recent studies showed that CPB binds to intestinal vascular endothelial cells during acute infection, leading to thrombosis and intestinal necrosis characteristic of CPB-mediated disease; whether the toxin binds to enterocytes is less clear. Once bound, CPB monomers rapidly oligomerize into hexameric or heptameric rings on the host cell surface, forming a pre-pore. Pre-pore formation is rapidly followed by toxin insertion into the membrane, presumably by an amphipathic transmembrane domain, resulting in cellular swelling and lysis. CPB is a potent toxin, with a calculated LD50 of 400 ng/kg (Table 5.2). The importance of CPB in mediating intestinal disease has been demonstrated using purified toxin challenges, as well as by toxin neutralization and genetic knockout approaches. Early studies utilizing monoclonal antibodies demonstrated that neutralization of CPB was necessary and sufficient to protect mice from lethal IV challenge with culture supernatants from type C strains. Better mimicking type C disease, which originates in and involves the intestines, later studies demonstrated that challenge with highly purified CPB mixed with trypsin inhibitor caused intestinal lesions in rabbit intestinal loops. Furthermore, pre-incubation of the CPB with a CPB-neutralizing monoclonal antibody eliminated this pathological effect. More recently, compelling evidence was provided by fulfilling molecular Koch’s postulates. In rabbit intestinal loops, PFO or CPA null mutants of type C strain CN3685 (a CPA/CPB/PFO positive strain) retained full pathogenicity. In contrast, a CPB null mutant was completely attenuated in this animal model. Furthermore, reversal of this CPB mutation restored virulence to wild-type levels. These findings provide solid evidence for the importance of CPB in mediating type C intestinal disease. The cpb gene is present on large plasmids, ranging from 65–110 kb in size, which may also encode other clostridial toxins such as CPE or TpeL. These CPB plasmids carry a tcp region, indicating their potential conjugative transfer ability. IS1151 sequences are also associated with the plasmid-borne cpb gene and those insertion sequences can excise the cpb gene from plasmids to form small circular DNAs that could be transposition intermediates. Collectively, these findings suggest that the cpb gene is highly mobile due to its apparent association with transposons and conjugative plasmids. Investigations into the regulatory mechanisms controlling CPB expression have shown that the VirR/VirS TCRS system controls CPB production. This regulation has pathogenic importance, since an isogenic VirR null mutant of CN3685 was fully attenuated in rabbit intestinal loops and complementation of that mutant to regain VirS/VirR expression also restored virulence. Western blotting of intestinal fluid detected no CPB in loops treated with the VirS/VirR null mutant, confirming the role of this TCRS in in vivo CPB regulation. Furthermore, when tested for lethality in the mouse enterotoxemia model, a significant decrease in lethality was observed in mice challenged with the VirR null mutant. The Agr-like quorum-sensing system also regulates CPB production, since an AgrB null mutant of CN3685 produced significantly less CPB in vitro compared to the wild-type parent. When tested in the rabbit model of necrotic enteritis, significant attenuation of the AgrB mutant was observed and this could be reversed by complementation to restore AgrB production. Furthermore, Western blot analysis of in vivo intestinal fluid samples demonstrated loss of CPB production by the AgrB null mutant, confirming that the Agr-like QS system regulates CPB production in vivo. Lastly, it was also shown recently that close contact of type C strains with host cells induces a rapid upregulation of CPB production. This effect requires both a functional VirS/VirR TCRS and the Agr-like QS system. C. perfringens type B and D strains secrete a relatively inactive ~33 kDa single polypeptide prototoxin that is proteolytically activated, creating the mature, ~29 kDa epsilon toxin (ETX). This activation can be caused by trypsin, chymotrypsin, or, possibly, C. perfringens lambda toxin in the intestine, and removes both N- and C-terminal amino acids from the prototoxin, but it is removal of the C-terminal amino acids that is critical for ETX activation. Once activated, ETX is the third most potent of all clostridial toxins, with an LD50 of ~100 ng/kg that ranks it behind only the botulinum and tetanus toxins. ETX belongs structurally to the aerolysin family of pore-forming toxins. The mature ETX protein consists of three domains. Domain I is thought to be the region interacting with receptors on host cells, while Domain II contains an amphipathic loop that inserts into membranes during pore formation. The first step in ETX action involves the binding of this toxin to a receptor. The identity of this receptor(s) is not yet clear, although hepatitis A virus cellular receptor 1 (HAVCR-1) has some properties expected of an ETX receptor. Once bound, ETX uses lipid rafts and caveolins to oligomerize into a heptameric pre-pore on the host cell membrane surface. It then extends a β-hairpin loop into the lipid bilayer to form an active pore. This pore formation results in a rapid decrease in cytoplasmic K+ levels, with a corresponding influx of Na+ and Cl− into these cells (Figure 5.1). The rapid loss of K+ is believed to cause cellular necrosis involving a rapid decrease in nucleus size without any DNA fragmentation. This process is not yet fully understood but involves ATP depletion. However, methyl beta cyclodextrin reportedly prevents pore formation but not cell necrosis, suggesting additional processes beyond pore formation may contribute to ETX-induced cytotoxicity. During infection, ETX prototoxin is produced by type B or D strains growing in the intestines. After its activation by intestinal proteases, as discussed earlier, or possibly C. perfringens lambda toxin, active ETX can affect the intestines (in goats) or it can be absorbed into the circulation (goats, sheep, and mice). The ability of ETX to traverse the intestinal epithelium even in the absence of intestinal damage suggests it may exploit paracellular permeability routes to reach the circulation. Once present in the circulation, ETX binds to internal organs, including the brain and kidneys. In the brain, ETX binds to endothelial cells and then alters the blood–brain barrier, which leads to the hallmark lesion of ETX brain effects; that is, perivascular edema that can develop rapidly. In animals with sub-acute and chronic disease, the brain lesions become necrotic and hemorrhagic and are known as focal symmetrical encephalomalacia. Another important brain effect of ETX involves increasing release of the stimulatory neurotransmitter glutamate. This effect is considered the major cause of neurologic symptoms observed during type B or D infections. Insertion sequences are located adjacent to the etx gene in both type B and D strains and those sequences can excise the etx gene from plasmids to form small circular DNAs that could be transposition intermediates. It is possible that those putative transposons may mobilize the etx gene to move between plasmids in C. perfringens. This etx mobilization could help to explain the diversity of etx plasmids detected amongst type D strains. However, only a single etx plasmid is present in type B strains, which could reflect plasmid incompatibility issues – in other words, perhaps only certain combinations of etx plasmids and cpb plasmids can be stably maintained together in type B strains. ETX is produced during log-phase growth. The regulation of ETX production is not well understood, despite some recent progress in this area. Contact with host Caco-2 cells was shown to upregulate ETX production and the Agr-like QS system is necessary for this host-cell-induced increase in ETX production by type D strain CN3718. However, ETX production is independent of the Agr-like QS system in two type B strains, indicating strain-to-strain (and perhaps type-to-type) differences in ETX regulatory control. Interestingly, the VirS/VirR TCRS does not appear to control ETX production by CN3718, suggesting there is not always regulatory cross-talk between the Agr-like QS and the VirS/VirR systems. Very recently, the CodY protein has been identified as a global regulator of virulence-associated properties of CN3718, including ETX production and bacterial adhesion to host cells. Iota toxin (ITX) has several distinguishing properties amongst C. perfringens toxins. First, ITX is one of only two toxins made by this bacterium (TpeL is the other) that clearly acts intracellularly. Second, ITX is the only C. perfringens toxin comprised of more than one polypeptide. ITX belongs to the clostridial binary toxin family because it consists of two polypeptides named IA and IB. Other members of this family include CDT of Clostridium difficile, the iota-like toxin of Clostridium spiroforme, and C2 toxin of C. botulinum. Both the IA and IB components of ITX are produced as propeptides that are proteolytically activated by intestinal enzymes or, perhaps, C. perfringens lambda toxin. Once activated, the ~74 kDa IB binds as a monomer to receptors on host cells. Recent reports indicate that IB interacts with the lipolysis-stimulated lipoprotein (LSR), which is also a receptor for C. difficile and C. spiroforme binary toxins (although LSR is not a C2 toxin receptor). The presence of multifunctional mammalian surface protein CD44 also reportedly increases ITX binding, so CD44 could be a second receptor or a co-receptor for ITX. Once bound to host cells, IB monomers use lipid rafts to oligomerize into a heptamer. This heptamer then binds the ~48 kDa IA, followed by internalization of the entire ITX into cells by endocytosis. IA escapes from the early endosome and enters the cytoplasm, where it ADP ribosylates actin at residue Arg-177, causing a collapse of the cytoskeleton and cell death (Figure 5.1). ITX is produced by the iap and ipb genes, which are co-transcribed in an operon and present on very large plasmids. Those plasmid-borne ITX genes are located near IS1151 sequences and either functional or silent cpe gene sequences. The ITX plasmids carry a tcp region, suggesting that they are conjugative. In addition, IS1151 sequences are associated with the iap/ibp genes, leading to the suggestion that the ITX genes have inserted onto cpe plasmids, converting progenitor isolates from type A to type E. The involvement of ITX in disease remains unclear, although type E strains have been associated with enterotoxemias in calves, lambs, and, perhaps, rabbits.
Toxins of Clostridium perfringens
Introduction
Type
Toxin production
α
β
ε
ι
A
+
−
−
−
B
+
+
+
−
C
+
+
−
−
D
+
−
+
−
E
+
−
−
+
A – Major toxins
C. perfringens alpha toxin
CPA
CPB
ETX
ITX
PFO
CPE
CPB2
NetB
TpeL
Molecular weight
42.5 kDa
35 kDa
29 kDa
Ia: 47.5 kDa
Ib: 71.5 kDa
54 kDa
35 kDa
28 kDa
33 kDa
~206 kDa
LD50 (in mice)1
3 µg/kg
400 ng/kg
100 ng/kg
Ia: 620 µg/kg
Ib: 940 µg/kg
13–16 µg/kg
140 µg/kg
ND
ND
11 mg/kg2
Primary disease
Myo-necrosis
Necrotizing enteritis
Entero-toxemia
Enteritis
Synergism with CPA; no primary disease
Enteritis
Unclear; possible entero-colitis
Avian necrotic enteritis
Possible role in avian necrotic enteritis
Trypsin sensitivity
Sensitive
Sensitive
Resistant (activated)
Resistant (activated)
Susceptible
Resistant
Sensitive
Unknown
Unknown
C. perfringens beta toxin
C. perfringens epsilon toxin
Iota toxin
B – Other toxins
C. perfringens enterotoxin
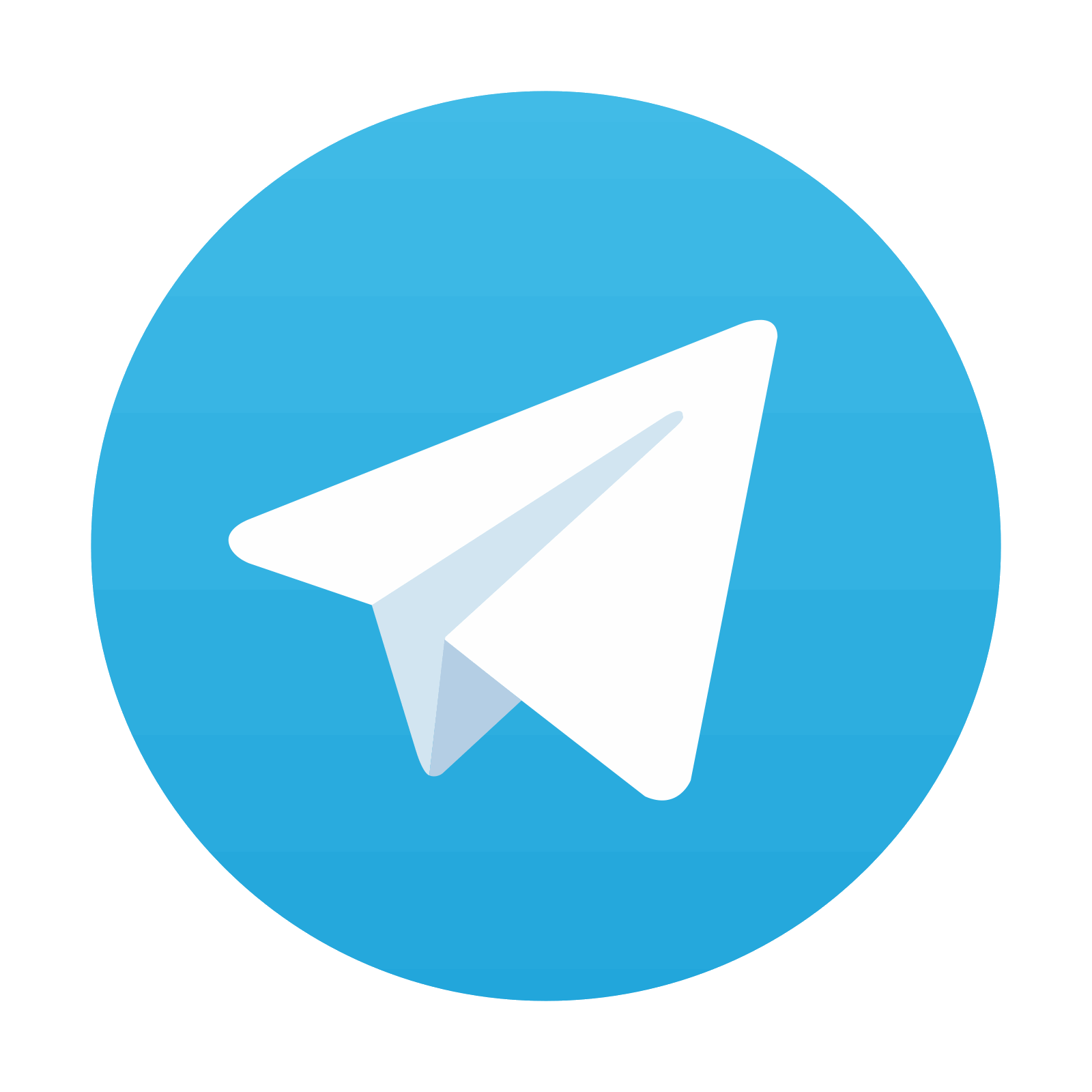
Stay updated, free articles. Join our Telegram channel
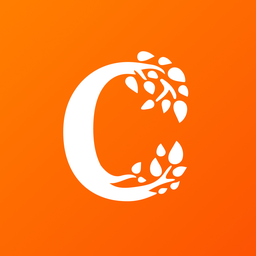
Full access? Get Clinical Tree
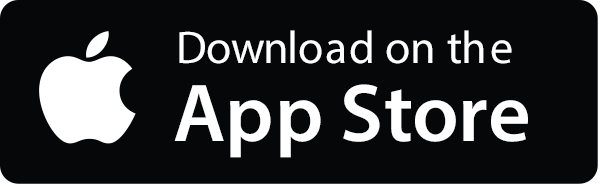
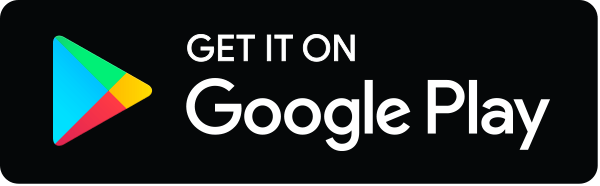