Fig. 5.1
The upper eyelid from a control rabbit has diffuse infiltrates of lymphocytes and plasma cells in the dermis as a spontaneous change. H&E. 5× objective
Neoplasms may arise from any of the structures in the eyelid, including the skin. Neoplasms reported in rats include squamous papilloma, squamous cell carcinoma, schwannoma, melanoma, granular cell tumor, basal cell tumor, sebaceous adenoma, malignant fibrous histiocytoma, and sarcoma [49].
Several drugs and chemicals can cause alterations involving the eyelids [50]. Edema of the eyelid can occur from systemic administration of iminodipropionitrile to monkeys.
Polychlorinated biphenyl (PCB) poisoning produces a chloracne condition in humans and affects sebaceous glands including the meibomian gland [51]. In monkeys, PCBs and similar compounds cause swelling of eyelids with meibomian gland hypersecretion and abnormal pigmentation of the conjunctiva. Microscopically, meibomian glands often contain keratin cysts with atrophy of the glands, increased numbers of layers of epithelial cells in the ducts, dilated ducts, and increased mitotic figures in basal cells [52–56].
Chronic application of topical epinephrine [57] to rabbit eyes results in a syndrome that has been called meibomian gland dysfunction. Features include plugging of the orifice of glands, microcysts, and opacification and enlargement of the gland. Ducts have increased stratification and keratinization, while the lumen is dilated with retention of desquamated epithelium.
Retinoids have been recognized to have effects on the meibomian gland. Isotretinoin (13-cis-retinoic acid), a therapy for acne vulgaris and keratinizing dermatoses, has been associated with side effects of blepharoconjunctivitis in 20–45% of human patients [58]. Systemic treatment of adult New Zealand white rabbits produces mild conjunctival erythema and crusting of the eyelid margins [58, 59]. These correlate microscopically with thickened meibomian gland ducts and ductal epithelium. The amount of acinar tissue is decreased due to degeneration and necrosis of acinar cells and accompanied by periacinar fibrosis. Additional changes include accentuation of the basal cells and decreases in basaloid cells lining acini with a lack of inflammation [58, 59].
Toxaphene administered orally to cynomolgus monkeys has been reported to produce local effects in the meibomian gland. Affected monkeys had inflammation, enlargement, or both, of the meibomian gland due to impacted diverticula in both the upper and lower lids causing accumulations of glandular secretions [60].
Systemic administration of small molecule inhibitors of epidermal growth factor receptor (EGFR) may produce granulomatous inflammation of the meibomian gland. This is suspected to be a pharmacologic effect due to a failure to secrete glandular content and continued production leading to subsequent glandular rupture. Release of glandular lipids into the surrounding tissue causes a granulomatous response.
Prostaglandin analogs (latanoprost, travoprost, and bimatoprost) are topically applied drugs that decrease intraocular pressure. In addition, there is lengthening of eyelashes (hypertrichosis) [61–64].
5.4.2 Extraocular Muscles
The extraocular muscles consist of six muscles (four rectus muscles and two oblique muscles) and, in species such as the dog and rabbit, the retractor bulbi [4, 7, 65]. The dorsal, ventral, and medial rectus muscles and the ventral oblique muscle are innervated by the oculomotor (III) nerve. The lateral rectus and retractor bulbi muscles are innervated by the abducens (VI) nerve. The dorsal oblique muscle is innervated by the trochlear (IV) nerve.
Extraocular muscles may be affected by light exposure and retrobulbar injections [66]. After 24 h of light exposure, extraocular muscles of rats were infiltrated with numerous neutrophils and mononuclear cells with myofiber degeneration. With time, neutrophilic infiltrates decreased and mononuclear cells persisted. Myoblasts formed to replace fragmented myofibers. Extraocular muscles may develop areas of inflammation (e.g., granulomas) associated with periorbital or retrobulbar injections and should be examined following these injections (Fig. 5.2).
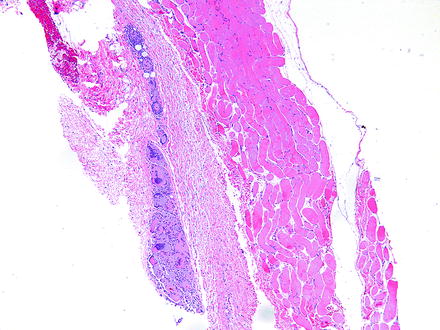
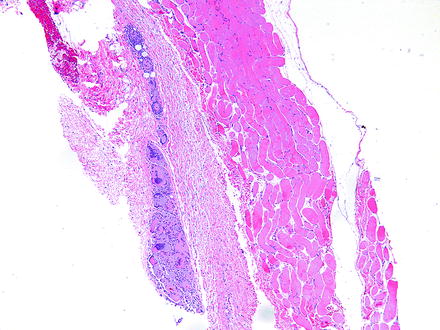
Fig. 5.2
Granuloma adjacent to an extraocular muscle in a rabbit given an injection into the periorbital soft tissues. Granuloma consists of numerous large, foamy, and eosinophilic macrophages, some of which are multinucleate. H&E. 5× objective
5.4.3 Orbital Glands
Except for the rodent’s harderian gland, most of the glands of the lacrimal system are not examined in routine toxicology studies but will often be examined in ocular toxicology studies. Since lacrimal glands and lacrimal structures may vary among different species, such as some species having the gland of the third eyelid and dogs having orbital glands that are not lacrimal glands (i.e., zygomatic salivary gland in the dog), it is important to be aware of these differences so that personnel involved in the collection of lacrimal structures can locate the intended structures [2–4, 7, 67–71].
Rodents have intraorbital and extraorbital lacrimal glands and a harderian gland [7, 68]. Cytomegaly and karyomegaly, along with nuclear pseudoinclusions, are features identified in the glands with males having a greater degree of these features than females. These differences may be explained by differences in gene expression in males versus females that may be associated with sex steroids [72].
Harderian glands are tubuloalveolar, merocrine glands without an intraglandular duct system [69, 70]. Harderian glands are usually larger in females than males and lymphoid cells may be present. The lipid-containing secretion contains porphyrins, mainly protoporphyrin IX, which may be a potential photosensitizer when activated by ultraviolet and blue components of daylight. The lumina may contain brown accretions which accumulate with age and may be associated with atrophy, granulomatous inflammation, and sclerosis. The amount of porphyrin in the gland is affected by castration, administration of androgens or estrogens, and nutritional deficiencies of riboflavin or vitamin A [69, 73, 74].
In the rabbit, the Harder’s gland may be referred to as a harderian gland but is different in gross and microscopic appearance than the harderian gland of rodents and does not produce porphyrins. The Harder’s gland is grossly composed of a white lobe and a pink lobe. The white lobe has smaller lumina and the cytoplasm contains larger lipid droplets. The pink lobe has larger lumina and stains more intensely with hematoxylin and eosin stain (Fig. 5.3). Small foci of lymphocytes are found sporadically in the lacrimal and Harder’s glands in rabbits [68].
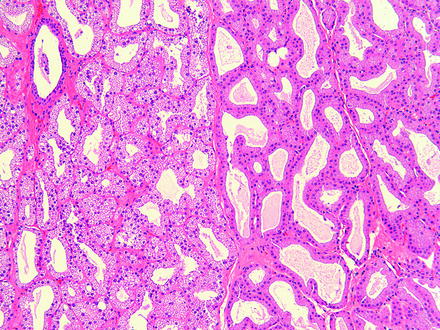
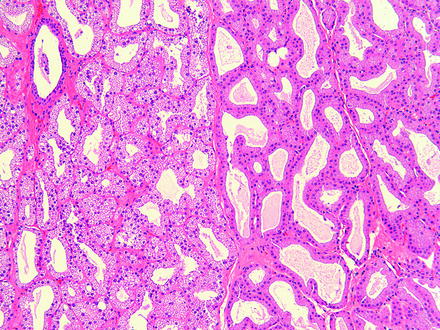
Fig. 5.3
Normal rabbit Harder’s gland. The adjacent pink (left) and white (right) lobes are readily distinguishable based on morphology. H&E. 10× objective
The main effect resulting from alterations in lacrimal glands is increased secretion (i.e., dacryorrhea) or decreased secretion leading to KCS. The morphologic changes that may be observed in lacrimal glands following injury are relatively limited. These include degeneration, necrosis (either oncotic or apoptotic), hypertrophy, atrophy, inflammation, and pigment accumulations. Focal lacrimal glandular hyperplasia, diffuse hyperplasia, or squamous metaplasia of ductal epithelium in rats may occur as a regenerative response to glandular damage [75]. Squamous metaplasia may be observed infrequently in the nictitating gland in dogs (Fig. 5.4).
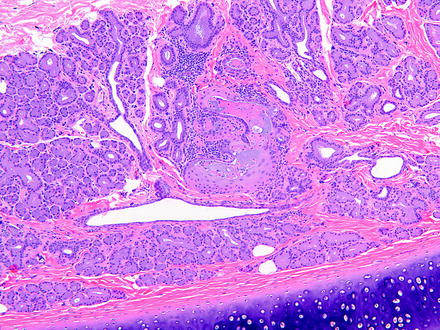
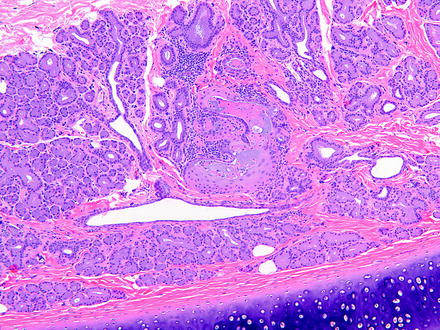
Fig. 5.4
Gland of the third eyelid from a control dog. The gland has focal squamous metaplasia. H&E. 10× objective
Harderian gland alteration (i.e., metaplasia), or harderianization, of the lacrimal gland is characterized as normal-appearing harderian acini in an otherwise normal lacrimal gland. Harderianization occurs as early as 3 weeks and increases with age and in the glands of both sexes, but males tend to have a greater incidence and extent of the change than females. The relative occurrence has been shown to be decreased with castration [76, 77]. Lacrimal gland alteration also occurs in rabbits and is characterized as normal lacrimal gland acini in the Harder’s gland (Fig. 5.5).
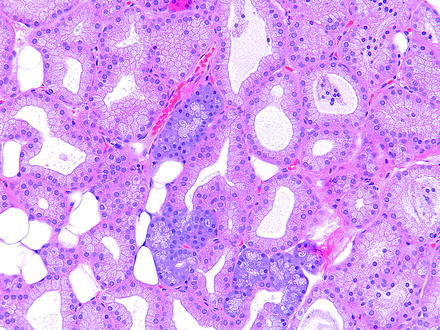
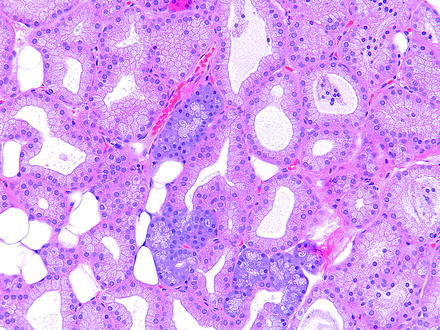
Fig. 5.5
Harder’s gland from a rabbit. Lacrimal gland alteration consisting of a cluster of basophilic acini morphologically similar to lacrimal gland acini located within the pink lobe of the Harder’s gland. H&E. 20× objective
Alterations of the lacrimal gland include minimal lymphoplasmacytic cell infiltration, especially in the rabbit, and focal glandular hyperplasia and squamous hyperplasia of ductal epithelium in rats [75, 76]. Hyperplasia generally occurs as a regenerative response to degeneration or inflammation [75]. Experimentally, diffuse acinar hyperplasia occurs in rats infused with recombinant human growth factor and is characterized by an increased number of cells per acinus, cell hypertrophy, anisokaryosis, accumulation of cell debris, and brown pigmentation [78].
Hyperplasia of the lacrimal gland may occur in aged rodents and appears to be more common in mice than aged rats or hamsters [49, 75, 79–81]. Hyperplasia of the lacrimal glands may be associated with degeneration and inflammation with squamous metaplasia of ducts [75].
In contrast to other periorbital tissues, lacrimal glands are sensitive to radiation injury. Radiation injury of ocular adnexa initially manifests acutely within 48 h of irradiation of the lacrimal glands. Degeneration and apoptosis or necrosis of the acinar cells occurs as a dose-dependent phenomenon. Ionizing radiation that exceeds 35Gy fractionated at 1.8–2.0 Gy (180–200 rad) per fraction increases the risk of lacrimal damage [82]. The ductular epithelium is also susceptible, but to a lesser degree than the acinar epithelium. Degenerating cells may have enlarged nuclei. Necrosis leads to a neutrophilic inflammatory response, and with time, there are decreased numbers of serous acini and atrophy of remaining serous acini. Periocular tissues will likely be edematous and diffusely infiltrated with neutrophils. However, this periocular tissue reaction may be due to vascular endothelial injury and not to glandular injury per se, as blood vessels are dilated and have hypertrophic endothelium [83, 84].
Drugs may affect lacrimal glands by decreasing lacrimal secretion resulting in KCS, but species susceptibility may vary [85]. For example, practolol, a ß-adrenergic receptor blocker, induces adverse ocular effects in humans, but not in animal models [86].
Lacrimal glands of dogs are affected by treatment with sulfonamide and 5-aminosalicylic acid [85, 87]. Sulfonamides have been reported to produce decreased tear production and subsequent KCS in dogs. The glands have chronic inflammation with atrophy and replacement by fibrous connective tissue, plasma cells, and lymphocytes. Atrophy of the lacrimal glands may occur in dogs following administration of 5-aminosalicylic acid for 1 year [88]. Atrophy and lymphoid cell infiltration occurs in the lacrimal glands, the glands of the nictitating membrane, and the parotid salivary glands. These dogs develop KCS with flattening and desquamation of superficial corneal epithelial cells, stunting and loss of cell surface microplicae, decreased density of cell cytoplasm, and disruption of anterior cell membranes. Corneal changes include inflammation, vascularization, and proliferation of fibroblasts. However, KCS does not appear to have been reported in human patients chronically treated with 5-aminosalicylic acid.
Inflammatory reactions in lacrimal glands can also be stimulated by various cytokines. These experiments have largely been for the purpose of developing models of autoimmune dacryoadenitis. Interleukin-1 injected into the lacrimal gland inhibits neural- and agonist-induced protein secretion with decreased tear output [89]. Associated with this is a severe inflammatory response that is reversible within 7–13 days after injection. The inflammation leads to destruction of lacrimal gland acinar epithelium. During resolution, there is increased proliferation of acinar and ductal epithelium with ultimate recovery to normal tear production. Interleukin-12 and interleukin-18 will also result in injury to the lacrimal gland, but without infiltrates of inflammatory cells [90]. Injection of these cytokines together via the intraperitoneal route results in apoptosis of serous acinar cells. Administration of either cytokine alone had no effects on these glands.
Another change in lacrimal glands is discoloration due to an accumulation of pigments. 7-Acetyl-1,1,3,4,4,6-hexamethyl-1,2,3,4-tetrahydronaphthalene (AHTN) is a fragrance material in consumer products that resulted in a green discoloration of lacrimal gland in female rats, but not male rats [91]. Discoloration was also noted in the liver and lymph nodes with reversal of the discoloration occurring in the liver, but not lacrimal gland. No microscopic correlate to the green discoloration of the lacrimal gland was observed.
Practolol administration to dogs results in a macroscopic dark brown to black discoloration of the lacrimal glands [92]. Microscopically, fine, dark brown pigment granules are present in the apical or supranuclear cytoplasm of the serous acinar lacrimal epithelial cells. Ultrastructurally, these correspond to membrane-bound dense bodies. In another study, practolol administration to dogs resulted in decreased tear flow and lymphocytic infiltrates into the lacrimal gland, but changes did not occur when a related compound (carteolol) was administered [85]. Phenazopyridine accumulates selectively in the lacrimal glands, nictitating glands, and glands of Moll, resulting in pigmentation [93]. Clinical effects include blepharospasm, photophobia, purulent conjunctivitis, and corneal ulceration, presumably from decreased tear production. Grossly, within 48 h of oral administration, phenazopyridine causes dark brown to black pigmentation of the lacrimal gland and gland of the third eyelid. Microscopically, brown to black pigment is visible in glandular acini within 48 h and accumulated with time. Neutrophilic infiltrates occur within 3–6 days of administration, and ultrastructurally, immature secretory granules containing phenazopyridine occur within 24 h as an osmiophilic material interspersed with normal granule constituents. With time, granules in acinar cells become pleomorphic or fuse.
Botulinum toxin B, a treatment for hypersecretion of lacrimal glands, blocks muscarinic receptors and when injected directly into the lacrimal gland suppresses lacrimation for 4–5 months in human patients. In mice, a similar physiologic effect can be produced, resulting in KCS, but without histologic changes in lacrimal glands [94, 95]. Similarly, administration of a muscarinic receptor agonist in rats results in corneal opacity with neovascularization [96]. Both lacrimal glands and harderian glands undergo hypertrophy which is suspected to be an adaptive response to the blockade. Diminution of lacrimal secretions is also observed with administration of other antispasmodic agents [97].
Common spontaneous microscopic findings of the Harder’s gland in rabbits include variability in the size of the lumina with scattered aggregates of dilated acini, minimal lymphoplasmacytic cell infiltrates, and focal areas of atrophy. Spontaneous findings involving the rodent harderian gland are common and include degeneration, especially in older animals, which may be characterized by atrophy of glandular acini, mineralization, fibrosis, and cyst formation. Additional spontaneous findings in harderian glands include inflammatory cell (neutrophils, lymphocytes, plasma cells) infiltrates, inflammation (dacryoadenitis), and excessive secretion of porphyrin (chromodacryorrhea).
Chromodacryorrhea may be caused by stress, necrosis or inflammation of the harderian gland, or administration of cholinergic drugs [98, 99]. Inflammation and necrosis may be caused by a viral disease (i.e., sialodacryoadenitis virus [SDV]) that occurs in young rats and mice [100]. The infection involves salivary glands, lacrimal glands, and harderian glands. The necrosis and edema of the harderian gland results in orbital swelling and proptosis of the globe which leads to corneal ulceration and keratitis. Other causes of necrosis, edema, and inflammation of the harderian gland include retro-orbital trauma from venipuncture, exposure to certain drugs (e.g., 4-chloronitrobenzene in rats), and exposure of albino rats to high-intensity light for 12–24 h (Fig. 5.6) [101–104]. Exposure of albino mice to constant light for as little as 24 h results in exophthalmos. Microscopically, the harderian gland has swollen secretory cells with an obliteration of glandular lumina. With time, glandular lumina contain lipid and cellular debris, and lining cells vary in appearance from columnar to squamous. Mitotic figures, leukocytes, and macrophages are present, along with necrosis of glandular cells and edema [102]. With cessation of the exposure, harderian gland changes regress within about a week.
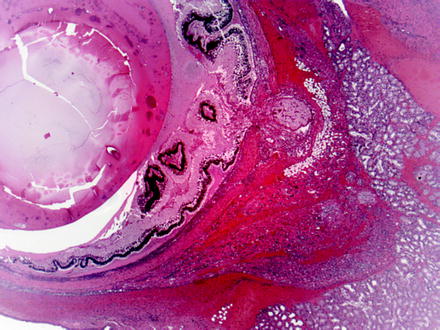
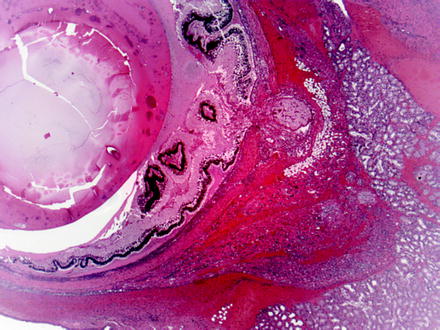
Fig. 5.6
Globe and adjacent tissues from a rat with iatrogenic trauma from retro-orbital blood collection. Multiple changes include necrosis, hemorrhage, and inflammation of periorbital tissues and intraocular exudation with serous retinal detachment. H&E. 2.5× objective
Inflammation of the harderian gland may also occur following exposure to exogenous chemicals.
Several agents, such as albuterol, have been shown to produce enlargement of the harderian gland [105]. Chronic aflatoxin administration to the Syrian hamster will result in hyperplasia and epithelial atypia of the harderian gland [106]. With time, some of these changes progress to papillary cystadenomas and solid adenomas. Recombinant human epidermal growth factor administered systemically has resulted in changes in the harderian gland of rats that include an increased numbers of epithelial cells per acinus, increased cell size, accumulation of cell debris in acinar lumina, and occasional areas of degenerating cells with pyknotic nuclei [78].
Atropine sulfate, a mydriatic often used in nonrodent species during ophthalmic examinations, causes a suppression of expulsion of secretory material [107]. Changes include dilation of acinar lumina and accumulations of brownish pigment. The increased brown pigment correlated with increased levels of porphyrin pigments in these glands. Glandular epithelial degeneration occurred in some animals which may have been associated with the prolonged accumulation of the porphyrin. The degeneration was characterized by thinning of the epithelium with formation of larger cytoplasmic vacuoles.
Many different agents and conditions cause effects in the harderian gland. Administration of the neuroleptic timipirone causes increases in the number of accretions of porphyrin in mice [108]. In rats, accumulation of porphyrin occurs with dietary pantothenic acid deficiency and results in hypertrophy of the harderian gland [109]. Atrophy of the harderian gland occurs following exposure to xenobiotics, and administration of retinoids causes a decrease in the weights of harderian glands due to a decrease in acini and small nuclei, indicating inactivity [110].
Since the harderian gland develops to a significant degree after birth, prenatal exposure to an agent (e.g., the herbicide 2,4-dichorophenyl-p-nitrophenyl ether) may result in degeneration after birth [111].
Spontaneous hyperplasia of the harderian gland may occur in aged rodents and appears to be more common in mice than aged rats or hamsters [49, 75, 79–81]. At times, hyperplasia of the harderian gland of the rat (i.e., Fischer 344) may be associated with degeneration, inflammation, and ductular squamous metaplasia [75].
5.4.4 Nictitating Membrane
The third eyelid (i.e., nictitating membrane, membrane nictitans) is a fold of conjunctiva that contains a cartilaginous plate surrounded ventromedially by a lacrimal gland (i.e., gland of the third eyelid, nictitans gland) which contributes to the production of tears [4, 112]. The inner conjunctival covering (i.e., bulbar conjunctiva) of the third eyelid contains numerous lymphoid nodules which can undergo lymphoid hyperplasia. The third eyelid is present in many laboratory animals (e.g., rabbits, dogs, minipigs) but is absent in rodents and primates. Retraction of the globe into the orbit by the retractor bulbi muscle or sinking of the globe into the orbit because of bulbar pain or a small size (e.g., microphthalmia or phthisis bulbi) allows the third eyelid to passively cover the cornea. Large red masses protruding from the third eyelid at the medial canthus may be the result of swelling and protrusion of the nictitating membrane [113, 114].
5.4.5 Lacrimal and Lymphoid Drainage
The products of the lacrimal glands flow over the cornea and are drained away via puncta in the eyelid margins that open into canaliculi and lacrimal sacs. The canaliculi travel a short distance vertically in the lid margin, then horizontally before connecting with the lacrimal sac. The canaliculi are lined by nonkeratinizing, stratified squamous epithelium. The lacrimal sac is located in the lacrimal fossa, a depression in the medial wall of the orbit. The lacrimal sac is lined by stratified columnar epithelium containing goblet cells. From the lacrimal sac, the nasolacrimal duct forms as a downward extension and can be histologically evaluated in appropriate nasal sections. The lymphatic drainage for the lacrimal glands and harderian glands is via periauricular and submandibular nodes in humans. Sampling of similar nodes in laboratory animal species should be considered when dosing via a topical route.
Treatment-related findings occur in the nasolacrimal duct. Hyperplasia of the lining epithelium of the nasolacrimal duct of rats has been noted following systemic treatment with recombinant human epidermal growth factor (EGF) [78]. Findings include a duct with an increased mucosal thickness, loss of cilia, and occasional pyknotic nuclei. EGF is secreted and cleared by the harderian gland and is eventually drained into the nasolacrimal duct. Interestingly, there was no corneal change, but corneal healing has been noted to be enhanced in cats and rabbits treated with EGF [115, 116].
Inflammation of the nasolacrimal duct is associated with chronic administration of antihistamines to rats and has been associated with the presence of stents [117, 118].
Hydrophilic coatings of the stents appear to decrease foreign body reactions and increase patency. Blockage of the nasolacrimal duct from inflammation or implanting a stent may result in epiphora (i.e., excessive tearing). Administration of docetaxel has been associated with this finding [119]. Cyalume, a chemiluminescent material, has been used to clinically evaluate the nasolacrimal system in human patients and does not injure the nasolacrimal duct but has resulted in findings in other locations [120].
5.4.6 Orbital Fascia and Other Orbital Contents
Orbital fascia consists of periorbita, Tenon’s capsule (i.e., fascia bulbi), and fascial sheaths of extraocular muscles [4, 7, 45]. Other structures include the retro-orbital sinus in mice and retro-orbital plexus in rats which are used for venipuncture.
An increase in the size of intraorbital contents results in an anterior protrusion of the globe (i.e., proptosis or exophthalmos). The cause is often the result of inflammation but could potentially be due to orbital neoplasia or the result of toxicity. In animals, orbital edema may be due to systemic administration of p-phenylenediamine and exophthalmos may be due to systemic administration of acetonitrile, aminocaproic acid, organic cyanides, or vitamin A [50].
5.4.7 Neoplasia of Lacrimal and Harderian Glands
Neoplasia of the harderian gland and lacrimal gland may occur in aged rodents and appears to be more common in mice than rats or hamsters [49, 75, 79–81]. Most tumors in rats are adenomas, with papillary, or cystic acinar differentiation, but orbital schwannoma has been reported [76]. The incidence depends on strain (e.g., Fischer 344, Osborne-Mendel and Wistar rats) but may be as much as 0.5–15% [81]. Harderian gland adenomas are frequently well-demarcated masses that compress the adjacent parenchyma, but usually do not have a well-defined capsule. They are papillary, cystic, or a combination and are composed of pseudoglandular structures and form arborizing and folded fingerlike fronds. Fronds are composed of a delicate fibrovascular core lined by cells that are usually tall columnar with foamy amphiphilic cytoplasm. The cells usually form a single layer, but areas are frequently present that give the appearance of having a basal layer of normal-appearing cells “capped” by smaller cells adjacent to the lumen. Harderian gland adenocarcinomas are usually well differentiated, differing from adenomas by greater atypia, invasion, and metastasis. Undifferentiated adenocarcinomas often contain a single large cytoplasmic vacuole, and mitotic figures are uncommon [121–123]. Harderian gland adenocarcinomas can metastasize to the eye and spread systemically [49, 76, 123]. Harderian gland tumors in mice may be induced by various agents, including ionizing radiation and genotoxic carcinogens [50, 81]. Harderian adenocarcinoma has been induced experimentally in rats by repeated injections with 10% urethane or when fed a low-fat diet containing 0.03% 2-acetylaminofluorene [49].
5.5 Cornea
The cornea is composed of several transparent layers that include (1) the precorneal tear film; (2) an outer, nonpigmented, nonkeratinized, and stratified squamous epithelial layer; (3) the anterior limiting membrane (i.e., Bowman’s layer) in primates; (4) an avascular stromal layer; (5) Descemet’s membrane; and (6) the corneal endothelium [4, 7, 124]. The function of the cornea is to transmit and refract light.
The results of iatrogenic intervention may result in various degrees of corneal opacity and should be distinguished from alterations due to toxicity. Some procedures such as surgery or an injection of a test article may affect all layers of the cornea. It is important to be aware of iatrogenic causes of corneal lesions that may be observed in the course of a toxicology study. Although most pathologists microscopically examine the eye in toxicology studies of systemically administered compounds, there are times when the test article is administered locally to the eye. In these studies, the pathologist needs to be aware of the route of administration and the alterations that may be present due to that route of administration. These effects are most notable when there is a surgical procedure or injection of the test article. Complications, or the lack thereof, should be documented. Corneal alterations that may be observed include an incision site and suture material as well as needle tracks from an injection. If the cornea is incised, complications may include inflammatory reactions to infected incision sites, down growth of corneal epithelium into the incision site, islands of corneal epithelium within the cornea that may become epithelial inclusion cysts (Fig. 5.7), corneal epithelial growth on the endothelial surface of the cornea, loss of corneal endothelium, deposition of foreign material into the eye or incision site or needle track (Fig. 5.8), and entrapment of the iris in the incision site (Fig. 5.9) [20]. For both incisions and needle tracks captured in histologic sections, the corneal stroma may be focally disorganized, which may or may not be sufficient to be observed as corneal opacity on ophthalmic or gross examination.
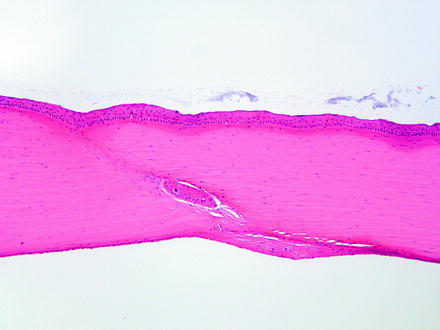
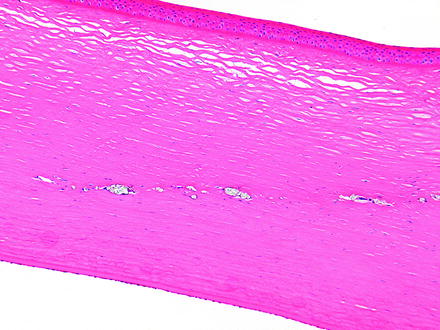
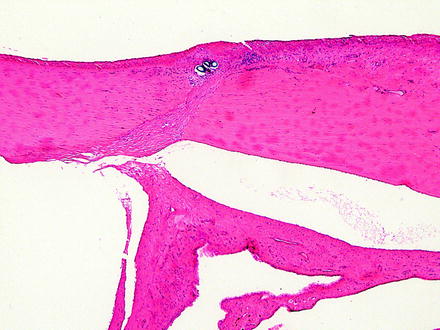
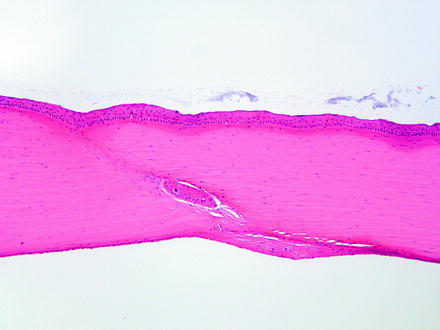
Fig. 5.7
Cornea from a rabbit two weeks following a surgical procedure. The incision site has a nest of corneal epithelium entrapped in the stroma, which, given sufficient time, may develop into a cyst. The endothelial surface has a focal area of retrocorneal membrane development. H&E. 10× objective
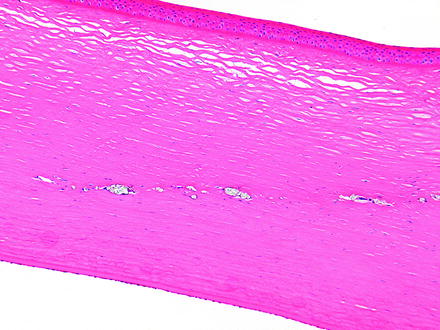
Fig. 5.8
Injection site in the cornea from a dog. There is a linear area of deposition of crystalline material from the injection remaining in the corneal stroma. H&E. 10× objective
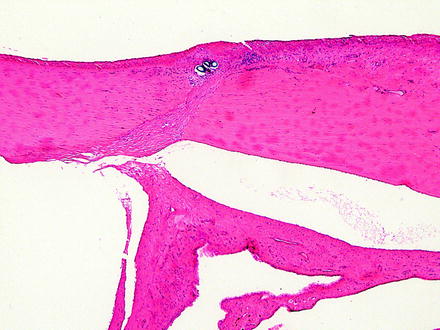
Fig. 5.9
Iridal entrapment in a rabbit. The iris is entrapped in a healing corneal incision. Note the suture cross sections near the corneal surface. H&E. 5× objective
In addition to iatrogenic findings, there are various alterations that occur spontaneously or in response to toxicity. Some changes may be localized to specific layers of the cornea, but more often than not, multiple layers of the cornea are affected. Toxic effects of the cornea have a tendency to affect either the outer cornea or the inner cornea. Toxic effects may be the result of a topical application or may be associated with systemic effects. Systemically administered drugs can also affect the cornea by secretion into the tear film (and therefore iritate the cornea directly), by injury of the lacrimal gland which removes the protective tear film which results in damage, or by inhibiting the protective corneal reflexes through depression of the central nervous system.
5.5.1 Macroscopic Corneal Opacities
Corneal opacities are noted on ophthalmic or macroscopic examination and refer to loss of transparency of the cornea. These are often due to mineralization, especially in rodents. Corneal opacities in rats may be diffuse or punctate and reversible or permanent. Diffuse corneal opacities occur in rats, especially males, and increase in incidence with age [125]. They may be punctate, focal lesions that give the cornea the dimpled gross appearance of an orange peel. Diffuse or focal opacities may be associated with or accompanied by conjunctivitis, keratitis, and corneal ulceration [126]. As such, corneal opacity is a nonspecific term that may correlate with multiple microscopic alterations.
In rats, corneal opacity as a spontaneous change may be unilateral or bilateral and tends to occur in males greater than or equal to 18 weeks of age. They correspond with multiple deposits of fine, white granules on the corneal surface grossly or deposits of basophilic fine granules or basophilic laminated plaques in the corneal basement membrane microscopically [127]. Corneal degeneration with superficial punctate opacities has been reported in Sprague-Dawley and Wistar rats of various ages and of both sexes [128]. Corneal opacities generally occur with an increased incidence with increased age in Sprague-Dawley rats [126, 127]. Others have reported reflective opacities in rats to be corneal crystalline deposits, with a higher incidence in males, that are usually bilateral, multifocal, and punctate, and usually in nasal locations oriented horizontally along the palpebral fissure [129].
Corneal abnormalities as spontaneous occurrences in mice on ophthalmic or gross examination have been reported in various strains [122, 126, 128, 130, 131]. Diffuse corneal opacities are infrequent in mice but increase in incidence with age and are due to mineralization of the anterior stroma [126]. Hubert reported corneal abnormalities in 4–5-week-old Swiss mice Crl:CD1(ICR)BR [46]. MRL mice with features of hyperparathyroidism have been described with corneal changes [132, 133]. Opacities in mice may be due to infrequent cage cleaning or cage environment, where urease-positive bacteria may increase ambient cage ammonia levels [130]. It has been suggested that some strains of mice may have a genetic predisposition. Therapeutic agents such as azathioprine and meticorten have been described as causing corneal opacities as well. In spontaneous diabetic KK mice, calcium is present as extracellular deposits of fine basophilic granules or as dense strips at the junction between the epithelium and the stroma, with older lesions tending to have neovascularization and minimal cellular infiltrates of neutrophils and mononuclear cells [132, 134].
5.5.2 Superficial Corneal Alterations
Changes in the corneal epithelium to superficial injury or toxicity include edema, erosions or abrasions, and ulcers from necrosis [135]. Grant characterizes the corneal changes from chemical burns from contact with liquids and solids; contact with gases, vapors, and dusts; or changes from systemic substances [50]. Chemical burns may cause immediate caustic injuries. Examples include alkalis and acids which not only affect the epithelium but also the stroma with edema and loss of mucopolysaccharides leading to opacification. The opacification is often accompanied by vascularization. Gases, vapors, and dusts may include a stinging sensation and result in tearing. Most substances that induce lacrimation can injure the cornea if the concentration is high enough. Findings include epithelial edema, vacuolation, delayed healing, or inflammation. Corneal epithelial edema is initially intracellular and appears as perinuclear pallor. With progression, there is pericellular fluid accumulation with the potential for bulla formation. An erosion (i.e., abrasion) is the partial desquamation of corneal epithelium and is usually due to focal mechanical trauma of a foreign body, such as dust particles. Since the defect does not extend past the corneal epithelial basement membrane, it may not be discernible on ophthalmic examination. With extension through the epithelial basement membrane (i.e., ulcer), the stroma will retain fluorescein. Ulceration may occur as a result of an initial abrasion with a bacterial infection. Abrasions are occasionally observed in the corneal epithelium in large animals [136]. Although in histologic section abrasions appear like small “craters” in the epithelium, they are likely instead linear. Microscopically, abrasions containing crowded cells in the basal layer are indicative of healing. Generally, the corneal epithelium heals quickly [137].
Hypertyrosinemia has been demonstrated to produce corneal opacities in laboratory animals [138–140]. Excessive tyrosine fed in the diet to rats produces initially an edema of the corneal epithelium with subsequent infiltrate of neutrophils into the superficial epithelial layers [139]. This progresses to neutrophilic infiltrates throughout the epithelium and eventually to infiltrates and edema of the corneal stroma. In rabbits, similar changes have been described with hypertyrosinemia with ophthalmic observations of pinpoint opacities and “glittering pre-endothelial structures” on slit lamp [138]. Microscopically, the corneal epithelium had focal proliferations with vacuolation of basal epithelial layers. Scattered epithelial cells underwent necrosis. Hypertyrosinemia induced by 2-(2-nitro-4-trifluoromethylbenzoyl)-cyclohexane-1,3-dione (NTBC), which is a potent inhibitor of 4-hydroxyphenylpyruvate dehydrogenase, produced corneal opacities in dogs, but not rats or rhesus monkeys [140]. All three species had elevations of serum tyrosine and aqueous tyrosine, indicating that there are some species-specific differences that lead to the corneal opacity with hypertyrosinemia.
Keratitis in various animal species is occasionally observed. These have been suspected to be due to environmental irritants such as ammonia and dusts from the bedding and feed, reduced lacrimal secretion, trauma, or exophthalmos [68, 130, 141]. Several strains of mice have had corneal opacities with histologic correlates of chronic inflammation of the corneal epithelium and anterior corneal stroma that included such features as ulcers and erosions, acute keratitis, neovascularization, and mineralization of the basement membrane [130]. These may be considered iatrogenic in the sense that they are an artifact of the test system, although animal colony management should reduce or eliminate these as a common occurrence.
5.5.3 Corneal Irritation or Local Toxicity
Adverse effects of drugs or ocular medical devices (i.e., contact lenses) on the outer cornea can be determined by use of the Draize ocular irritation scoring method and its various modifications [17, 142–146]. Currently, some of the features of a clinical adverse reaction are being predicted by use of in vitro or ex vivo assays such as the bovine corneal opacity and permeability assay (BCOP) [147, 148]. The BCOP will largely mirror in vivo responses, except for the absence of an inflammatory response or neovascularization in these in vitro and ex vivo assays.
The various responses to irritating substances such as alkalis, acids, and surfactants from direct contact to the cornea have been studied to better understand the pathology as well as to serve as benchmarks in the development of in vitro and ex vivo substitutes to in vivo testing [135, 149–154]. Alkali burns are caused by saponification of cell membranes, allowing greater penetration of the injurious chemical, making alkali burns usually the most severe injury. Acid burns are caused by coagulation and precipitation of cellular proteins, and the natural buffering of tissues tends to limit the injury of acids as compared to alkalis [151]. Surfactants generally produce a milder injury than acids or alkalis. Surfactants are broadly classified as cationic, anionic, or nonanionic based on their chemical structure, but cationic surfactants tend to produce the greatest injury due to the precipitation of cellular proteins [17]. Anionic surfactants are intermediate and tend to cause cell lysis, while nonionic surfactants do not produce protein precipitation or cell lysis [150]. Regardless of the nature of the chemical, they all can produce erosion, denudation, and necrosis of the corneal epithelium [135]. Greater irritant capacity generally corresponds with greater extent and depth of injury. Mild irritants are therefore confined to the epithelium, while moderate irritants will injure the corneal stroma, and severe irritants will affect the deep stroma and possibly the corneal endothelium. Affected corneal stroma will be edematous and have necrosis and lysis of keratocytes. The corneal endothelium may be lost. With time, the cornea will respond with keratocyte regeneration, neovascularization, conjunctivalization, or epidermalization of the corneal epithelium. The degree of response will reflect the initial depth and area of injury. It should be noted that although the corneal epithelium is first and often most greatly affected, this may not always be the case. For bleaching agents, it was noted that the corneal stromal injury was greater than the corneal epithelial injury [153]. Severe or perforating keratitis may lead to inflammation of the iris (i.e., iritis), anterior uvea (i.e., anterior uveitis), inner aspect of the globe (i.e., endophthalmitis), or the whole globe (i.e., panophthalmitis). With chronic inflammation affecting most or all of the globe, then shrinkage and distortion of the globe (i.e., phthisis bulbi) can occur.
The outer cornea may be injured due to desiccation from an acute or chronic loss of tear film. The tear film may be compromised by a lack of production or a lack of covering of the corneal surface. A lack of production may be due to inflammation of the lacrimal gland or administration of certain pharmacological compounds, including local anesthetics, anticholinergic compounds, or anticholinesterases, which decrease lacrimal secretions. Compounds that affect the lacrimal gland or meibomian glands may result in a minimal, reversible changes in the cornea (e.g., keratinization) or, if more severe, a chronic condition involving the cornea, KCS. Changes in the cornea associated with KCS include keratinization, epidermalization, pigmentation, fibrosis, and neovascularization of the superficial stroma and possibly mononuclear or mixed inflammatory cell infiltration.
Acute corneal desiccation may occur following general anesthesia as an iatrogenic cause of corneal changes [155, 156]. Desiccation in the interpalpebral area leading to keratoconjunctivitis sicca and associated secondary uveitis is reported in rats following general anesthesia with a combination of xylazine and ketamine [157]. Animals undergoing general anesthesia will often have incomplete closure of the eyes (lagophthalmos). Even if the anesthesia is not prolonged, desiccation of the areas of cornea not covered by the eyelids will occur by evaporation following breakup of the precorneal tear film, which can happen in seconds to minutes. In humans, the time required for injury is longer than in rodents, with no changes reported in human eyes up to approximately 100 min. In addition to lagophthalmos, general anesthesia may also reduce the production and the stability of tears [155]. Microscopically, corneal desiccation may result in changes of degeneration or erosion and thinning and ulceration of the corneal epithelium. Changes generally will be acute to subacute, and the changes of chronic KCS would not be anticipated as iatrogenic changes in laboratory animals. Several authors have described drug-induced corneal opacities related to narcotic analgesics or prolonged anesthesia [157–159]. For example, a single administration of the long-acting narcotic analgesic 1-alpha-acetylmethadol to male Sprague-Dawley rats resulted in localized corneal opacities mainly in central, nasal, or inferior-nasal regions after 3–5 days [159]. Microscopically, these animals had thickened corneal epithelium, loss of cellular polarity, hyalinization of the basement membrane, stromal vascularization, spindle cell proliferation, inflammation, and frank perforation. It was considered likely that this was due to adverse effects on corneal sensory innervation, blinking, or tear formation, instead of direct chemical effect on the corneal epithelium.
Although not due to lagophthalmos and drying, other anesthetic agents have been associated with corneal epithelial changes. These include administration of agents such as cocaine, tetracaine, and proparacaine. Chronic use of these anesthetics may alter the plasma membrane integrity of corneal epithelial cells, cause release of enzymes such as lactate dehydrogenase, and cause decreases in mitochondrial dehydrogenase activity [160]. Corneal opacities have been reported in rats following administration of morphine and narcotic analgesics [159]. Microscopically, there may be thinning of the corneal epithelium, and generally, these are acute or subacute and do not progress to the changes of chronic keratoconjunctivitis sicca.
Chronic desiccation of the cornea leading to KCS may also be the result of anterior displacement of the cornea. This may occur as the result of an enlarged globe (e.g., glaucoma) or from anterior displacement of the entire globe (exophthalmos, proptosis) secondary to orbital swelling. Inflammation of the harderian gland with chromodacryorrhea from repeated orbital bleeding or from a viral infection (e.g., sialodacryoadenitis) may also be causes [100]. Anterior displacement of the cornea appears to affect males more than females. Treatment with clonidine induces exophthalmos and a reduction in tear flow in mice and rats resulting in flattening and desquamation of corneal epithelium and vacuolation of basal cells. These changes may progress to keratoconjunctivitis and anterior uveitis [141].
In addition to irritants and loss of tear film, corneal epithelial lesions may be produced by drugs that inhibit mitosis and reduce the proliferative capacity of the corneal basal epithelium. A variety of antineoplastic agents are known to affect the cornea, and these often present clinically in patients as punctate keratitis [161]. For example, the antineoplastic drug, capecitabine, is converted to 5-fluorouracil in vivo and is concentrated in tears. Clinical signs in dogs include multiple erosions of the cornea with superficial corneal epithelial pigmentation and neovascularization [162]. Microscopically, the corneal epithelial basal cells were disorganized and had an abnormal morphology resulting in a thinned corneal epithelium. Thinning (i.e., atrophy) of the corneal epithelium with progression to ulceration and inflammation also occurred in dogs given inhibitors of oxidosqualene cyclase [163]. Atrophy has also been described in human patients treated with triparanol, a late stage blocker of cholesterol synthesis [164, 165]. Decreases or alterations in tear production may occur with oxidosqualene inhibitors related to reductions in the lipid-rich glands such as the meibomian glands [166].
Hyperplasia of the corneal epithelium may occur as a nonspecific response to injury such as to topical chemical injury as described above. Conjunctivalization is a metaplastic response where the corneal epithelium transforms into the appearance of conjunctiva, with goblet cell differentiation and irregular thickness. A chronic change observed in the corneal epithelium of mice is goblet cell metaplasia [167]. Hyperplasia has also been observed with systemically administered compounds, a notable example being EGF [168]. High doses of systemically administered recombinant EGF produced diffuse, uniform thickening of the corneal epithelium due to an increased number of layers of corneal epithelial cells overlying basal cells that were hypertrophic and hyperplastic.
Although uncommon, localized corneal proliferation may occur, especially in chronic studies. Corneal hyperplasia may be observed as a focal, diffuse, or nodular thickening of the corneal epithelial layer and is not considered to be preneoplastic [167]. Hyperplasia may be accompanied by keratinization. Corneal proliferation may include squamous cell hyperplasia, epithelial hyperplasia, squamous cell papilloma, and squamous cell carcinoma as chronic changes in rats. Squamous cell carcinoma is not known as a spontaneous finding in mice [169]. The corneal surface epithelium may be focally thickened due to congenital findings such as corneal dermoids. A corneal dermoid is the localized presence of tissue appearing as skin within the cornea. The finding may be unilateral or bilateral and will be discovered on prestudy ophthalmic examinations. These have been described in the rat, hairless and haired guinea pig, rabbit, and dog [170–174].
Squamous cell hyperplasia and acute keratitis have been induced in female HRA/Skh mice treated with 8-methoxypsoralen combined with UV radiation [175].
5.5.4 Superficial Corneal Stromal Alterations
Alterations in Bowman’s layer in primates include edema, spheroidal degeneration, or interruptions and may or may not be noted as corneal opacities. Edema of Bowman’s layer is usually associated with edema in the overlying epithelium or the underlying stroma and is manifested by decreased eosinophilia of this layer microscopically. Spheroidal degeneration of Bowman’s layer is considered collagen deposition and appears as extracellular spherical deposits. This change occurs as a sequela of chronic actinic exposure in humans. Interruptions in Bowman’s layer are often traumatic or related to surgery. Since Bowman’s layer does not regenerate, these interruptions will be filled in either with overlying corneal epithelium or with fibrous connective tissue from the underlying stroma.
5.5.4.1 Subepithelial Corneal Mineralization
Corneal mineralization can be due to multiple causes. Corneal dystrophy is a term commonly used in veterinary ophthalmic pathology to encompass many of these changes. However, corneal dystrophies are defined as spontaneously occurring, noninflammatory changes bilaterally affecting the central cornea with no associated systemic disease, when narrowly defined by the definitions used in human ophthalmology. Thus, narrowly defined, true corneal dystrophies as a primary disease appear to be rare in animals, while the more nonspecific calcific band keratopathies are relatively common. Calcific band keratopathy occurs in human patients in association with a variety of ocular and systemic diseases including chemical and physical injury, chronic glaucoma, and phthisis bulbi. It may also accompany hyperparathyroidism or vitamin D poisoning [176]. Hypercalcemia produces bilateral findings and may extend to include calcification of the bulbar conjunctiva [177]. In humans, it also usually involves Bowman’s layer.
Calcific band keratopathies, which as previously mentioned are also commonly referred to as corneal dystrophies in laboratory animals, are mineralized or calcified deposits presenting microscopically as basophilic granularity along the corneal epithelial basement membrane [178]. The extent and severity of secondary changes varies with animal strain, age, and experimental conditions [125]. The macroscopic lesion is often characterized by a central, bilateral, and elliptical band in the area underlying the palpebral fissure [179]. More advanced findings may consist of epithelial necrosis, foreign body reaction, and scarring. Often, experimental corneal calcification is the result of trauma, which causes edema and proliferation of fibroblasts. These cells synthesize and release glycosaminoglycans into damaged regions, where the calcification develops; the free anionic groups of glycosaminoglycans tend to bind calcium [180].
Drying of the cornea is an important mechanism of mineralization because evaporation of the tear film from the cornea results in supersaturation and precipitation of calcium in the superficial corneal stroma. Band keratopathy may develop due to inability to cover the underlying cornea with the lids, as well as high ammonia levels from urease-positive bacteria in the bedding [130]. In mice, it may be due to harderian gland adenoma leading to proptosis and keratitis.
A true corneal dystrophy has been described in the American Dutch belted rabbit and the New Zealand white rabbit. The American Dutch belted rabbit develops opacities involving the corneal epithelium, basement membrane, and superficial stroma that are noted clinically with the use of a slit lamp. Microscopically, the basement membrane is thickened, and the overlying epithelium is thinned and disorganized. The underlying corneal stroma is also often disorganized [181]. The corneal dystrophy reported in the New Zealand white rabbits is characterized by a normal basement membrane, but the overlying epithelium is irregular with both thickened and thinned areas [182]. Calcification or mineralization of the cornea independent of these corneal dystrophies has been reported in rabbits due to local trauma, hypervitaminosis D, and administration of dihydrotachysterol [158, 183–185]. Corneal injury potentiates the corneal calcification produced by the hypercalcemia of dihydrotachysterol in rabbits. Calcification also occurs in the rabbit cornea in response to immunogenic uveitis combined with hypercalcemia [186].
Experimental corneal calcification in the rabbit has been produced by mild irritation with carbon dioxide laser [183]. Ultrastructurally, numerous spherules of fine crystals were within the basement membrane of the corneal epithelium. Some calcific spherules were also present in membrane-bound vesicles within the cytoplasm of keratocytes. These deposits stained black with von Kossa silver stain and red with alizarin red S stain, indicating the presence of calcium. Additionally in rabbits, ––morphine appears to cause drying of the cornea [158] with development of exophthalmos in treated rabbits.
In rats, corneal mineralization, often referred to as corneal dystrophy, is a condition where calcium and phosphorus is deposited in the superficial cornea in a diffuse or punctate distribution along the palpebral fissure [179]. It is postulated that changes in the corneal microenvironment (e.g., pH or ion concentration) can cause calcium to precipitate [128, 177]. Strains of rats involved include Wistar, Sprague-Dawley, and Fischer 344 [122, 126, 128, 130, 178]. In Sprague-Dawley, Fischer 344, and Wistar rats, mineralization occurs more in males than females [129]. A syndrome called spontaneous corneal dystrophy has been described in Fischer 344 rats that have extracellular electron-dense deposits of calcium and phosphorus in basement membranes of corneal epithelial cells and other tissues (e.g., renal tubular basement) such that the condition is not strictly limited to the cornea and therefore does not fit the strict definition of corneal dystrophy used in human ophthalmology [178, 187]. Band keratopathy can occur in rats due to the increased dietary content of vitamin D3, local ocular disease, or systemic abnormalities in calcium-phosphate metabolism [131]. Excess vitamin D causes similar findings in the corneas of albino and pigmented guinea pigs. However, the guinea pig cornea appears to be much less susceptible to experimental corneal calcification than the rat or the rabbit.
In the rat, de-epithelialization of the central or entire cornea is effective in inducing corneal calcification. Other treatments in the rat, including isoproterenol, alloxan [188, 189], increased dietary vitamin D3 [185], subcutaneous administration of morphine sulfate, and suturing the eyelids open, all can be associated with corneal calcification.
Meador et al. described corneal mineralization associated with epicardial mineralization in SCID mice with deposition of mineralized material in the corneal basement membrane or in thin clefts at the epithelial/stromal junction [192]. In more severe instances, deposits were surrounded by macrophages. The corneal epithelium was variably thinned and dystrophic.
5.5.4.2 Corneal Pigmentation
There are many different causes of corneal pigmentation. Brown, hemosiderin-laden macrophages may be present in the anterior chamber and adherent to the endothelium or in the corneal stroma following hemorrhage; yellow particles in the anterior layers of the cornea have been reported in the human cornea following administration of compounds containing gold [191]; and pigmentation occurs following administration of minocycline, amiodarone, phenothiazines, antimalarials, and salts containing silver [192–194]. Pigmentation may also be a consequence of migration of melanocytes from the conjunctiva associated with neovascularization and reparative responses from previous ulceration.
5.5.4.3 Corneal Stromal Edema
Nonspecific alterations in the stroma include edema and inflammation. Stromal edema is characterized by increased thickness of the stroma due to increased interstitial fluid. Inflammatory cells may infiltrate as part of the edema process. Expansion of the stroma by edema disrupts the regular arrangement of the collagen bundles of the stroma, producing opacity. Proper histologic processing is important to appreciate corneal edema, as artifacts can lead to notable differences in corneal thickness as observed on histologic slides.
5.5.4.4 Corneal Inflammation
Corneal inflammation is almost always accompanied by edema. Inflammation first presents grossly as perilimbal hyperemia. With time, cells migrate into the corneal epithelium and stroma. When inflammation is severe, cells will migrate from the iris into the anterior chamber (hypopyon) and then into the cornea. Nonulcerative inflammation, such as superficial keratopathy, if minor, can heal without any residual opacity. Stromal keratopathy involves the stromal layer and will likely be vascularized. Ulcerative corneal inflammation usually has a bacterial component that follows the initial injury. Sequelae to keratopathy include scars, which microscopically appear as fibrosis and will be clinically opaque. Keratectasia (i.e., ectatic cicatrix), descemetocele, and adherent leukoma may form. Pterygium is conjunctival growth onto the cornea and should be distinguished from pseudopterygium which is the extension of a conjunctival flap over the cornea. Keratectasia (ectatic cicatrix), descemetocele, adherent leukoma, and corneal staphyloma are the more serious consequences of ulceration.
Keratitis and sequelae are often related to injury and increase with age, with the rat having a greater incidence of keratitis than the mouse [125, 126]. Keratitis has been associated with SDV and coagulase-positive Staphylococci [100, 125, 129]. Corneal scars, with superficial vascularization, may be attributed to prior trauma [129]. Large animals such as the dog and monkey tend to have only infrequent keratitis, and the incidence in the dog has been report to be as low as 0.5%. In dogs, this has been attributed to dust and bedding and may be exacerbated when ocular defense mechanisms are depressed following administration of pharmacological agents.
Keratitis may be a manifestation of photosensitization after exposure to phenothiazine, methoxsalen, or other photosensitizing compounds [17, 50]. Ultraviolet radiation exposure and phototoxicity have been demonstrated to occur in humans and a variety of animal species with the cornea being one site of injury [161]. UV radiation has been associated with marked loss of keratocytes in mice [195]. Phototoxicity following exposure to Penicillium viridicatum culture material includes epithelial changes in the cornea, such as ballooning degeneration, proliferation and thickening of the corneal epithelium, and thinning of the corneal stroma [196]. Changes in the corneal stroma include corneal vascularization, fibrosis, and inflammation that may lead to keratoconus and rupture of the globe. The observed thinning of the cornea with UV light and the thickening demonstrated with some photosensitizers suggest that there may be effects related to differences in the physical energy injury.
5.5.4.5 Corneal Neovascularization
Neovascularization is the vascularization of a normally avascular corneal stroma (Fig. 5.10). It is a common response to corneal injury and is often associated with inflammatory mediators [197, 198]. Corneal neovascularization may be observed in nude or hairless mice [167, 199]. Blood vessels interfere with transparency by their physical presence and participate in inflammation. Vascularization of the cornea is a beneficial repair response to inflammation, although, as a sequela, it may produce opacities due to the persistence of nonperfused ghost vessels visible on ophthalmic examination as linear opacities. Vascularization will be confined only to the injured cornea and does not generally involve healthy cornea. Findings often associated with corneal neovascularization include conjunctival congestion, corneal stromal edema, and inflammation.
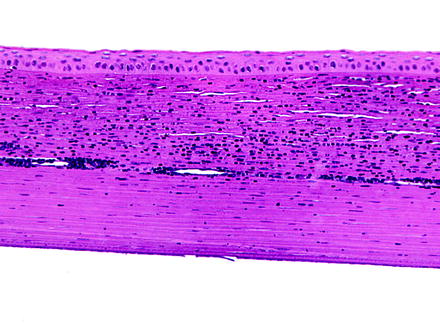
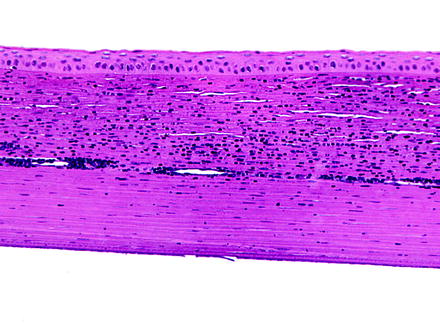
Fig. 5.10
Cornea from a rabbit. The cornea is infiltrate by numerous inflammatory cells, and a relatively large vessel extends laterally through the corneal stroma. H&E. 20× objective
Neovascularization has also been associated with deficiencies of tryptophan, riboflavin, vitamin A, and zinc [200, 201]. Some compounds can induce neovascularization, such as EP4-prostaglandin E2 agonists. When this compound was administered topically, corneal neovascularization was induced as if either through a pharmacologic or inflammatory mechanism [202]. The induction of neovascularization may be inhibited by administration of compounds such as anti-vascular endothelial growth factor (VEGF) antibodies [203].
Not all corneal stromal vascularization is neovascularization. Limbal vessels can occasionally extend a short distance into the peripheral cornea. This finding is observed in the corneas of rabbits with no other corneal or limbal changes and is considered to represent normal finding. Careful examination of multiple sections of the eye from control animals can help differentiate normal variability from abnormality. In addition, corneal nerves may occasionally be observed within sections and should not be confused with neovascularization (Fig. 5.11).
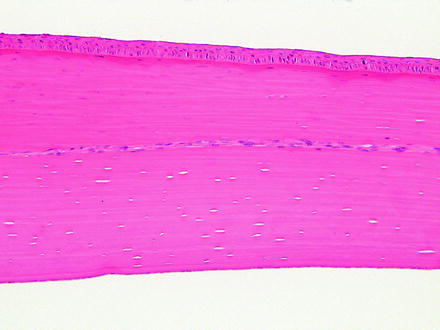
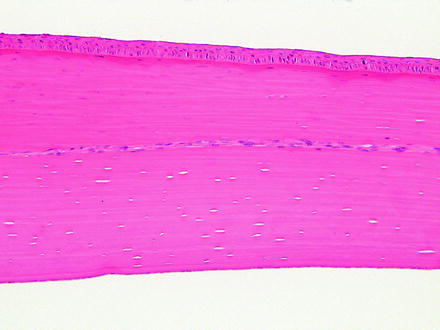
Fig. 5.11
Cornea from a rabbit. A nerve traverses laterally through the corneal stroma and is occasionally observed as a normal structure. H&E. 20× objective
5.5.4.6 Lipid Keratopathy and Corneal Phospholipidosis
Corneal lipidosis can be observed on occasion as either a spontaneous or induced change. Lipid deposition in the cornea of rabbits and dogs has been observed in animal colonies. Rabbits with altered lipid metabolism, notably the Watanabe WHHL rabbit or rabbits on high-fat diets, are susceptible to corneal lipidosis [204–207]. The eyes have grossly visible corneal opacities, initially as clouding of the medial ventral cornea, which will progress to pearly corneal opacities extending to the central and lateral cornea. Microscopically, the anterior corneal stroma has infiltrates of cells with necrosis and cellular infiltrates. Infiltrates consisted of large, pale, and foamy macrophages. Foci of necrosis contained Sudan black-positive materials. Corneal lipidosis may also be drug induced, with birefringent crystals in outer stroma that are visible with polarized light and stain with Sudan black. The guinea pig also may present with lipid deposition as part of a disease process including conjunctivitis, keratitis, and keratoconjunctivitis sicca [208].
In dogs, corneal lipidosis is a rare spontaneous change but when it occurs may present as marginal, zonal, or epithelial forms [209]. In beagle dogs on life span toxicology studies of radionuclides, similar corneal opacities have been observed with microscopic changes of amorphous to crystalline lipid deposits in the anterior cornea [209]. These appeared to be a spontaneous change in aged dogs, as no evidence of a systemic hypercholesterolemia was detected. However, deposits were determined to be neutral fats, phospholipids, and cholesterol [210].
Cationic amphiphilic drugs are recognized to produce phospholipidosis of the cornea. Chloroquine, tamoxifen, and other drugs in humans produce corneal deposits characterized by lipid in lysosomes of the corneal epithelium and keratocytes [161, 211–213]. In humans, the phospholipidosis is reversible and associated with little or no visual impairment [191]. Corneal phospholipidosis is also described in rats and dogs [214–216] and could occur in any species dosed with cationic amphiphilic compounds. Histologically, phospholipidosis in the cornea appears as clear lipidosis-like alterations in the corneal epithelial cells and keratocytes with bluish granules with hematoxylin and eosin staining. Semithin sections demonstrate densely staining toluidine blue positive, irregular cytoplasmic inclusions [215]. Transmission electron microscopy shows the typical lamellar and crystalline-like inclusions. Tilorone, an immunostimulatory agent that causes increased production of interferon, has been shown to produce both phospholipidosis and mucopolysaccharidosis in rats. The phospholipidosis is typical and reversible, while the mucopolysaccharidosis occurs as deposits of glycosaminoglycans in lysosomes of the corneal epithelium and keratocytes which persist for long periods [217, 218].
5.5.5 Deep Corneal Alterations
Descemet’s membrane undergoes changes secondary to changes in the overlying corneal stroma or underlying corneal endothelium. With expansion of the corneal stroma, such as with edema, Descemet’s membrane may develop tears (i.e., stria), and the edges of large tears in Descemet’s membrane often coil when examined microscopically (Fig. 5.12). Deep ulceration of the corneal stroma may result in anterior displacement of Descemet’s membrane into the stromal defect forming a descemetocele. Since Descemet’s membrane is continuously produced by corneal endothelium, it will become thickened with age or can undergo duplication [219]. Degenerative changes in Descemet’s membrane will manifest as irregular or diffuse thickening and are generally due to disease of the subjacent corneal endothelium. Descemet’s membrane may extend onto structures of the filtration angle (i.e., descemetization), and cellular material may be embedded within it as a result of transcorneal injections.
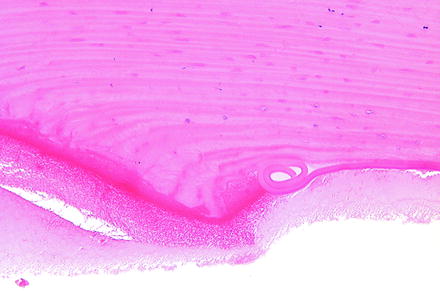
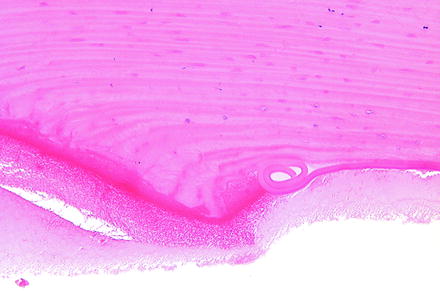
Fig. 5.12
Cornea from a rabbit 1 day following a surgical procedure. The incision site (left) has coiling of Descemet’s membrane (right). H&E. 20× objective
Degeneration or loss of the corneal endothelium, with sufficient time, will result in irregular thickening of Descemet’s membrane. Since Descemet’s membrane is continuously laid down during the life of an animal, where the endothelium is healthy and active, Descemet’s membrane will continue to be expanded. In those areas where the endothelium is not actively laying down Descemet’s membrane, it gives the impression of local thinning, which is only a relative change.
The corneal endothelium is important in maintaining fluid balance within the corneal stroma, so a morphologic or functional loss of corneal endothelial cells results in diffuse corneal edema and opacification. When the inhibition is the result of a compound, the resulting opacity is often diffuse in comparison to localized injury. Intracameral implantation of medical devices can lead to a physical loss of the endothelium and may demonstrate this localized opacity. Compounds that are known to injure the corneal endothelium include 5-fluorouracil, local anesthetics, methylene blue, and tobramycin. Compounds such as methylhydrazine, 1,2-dichloroethane, 1,2-dibromoethane, and 1,2-dichloroethylene have been reported to cause corneal endothelial cell injury in animal studies, but not in humans [50]. With sufficient time, loss of the corneal endothelial cells causes irregular thinning of Descemet’s membrane from a lack of continuous deposition and normal thickening.
The corneal endothelium has limited regenerative capacity which does vary some by species. For example, endothelial cells of nonhuman primates, dogs, and cats enlarge and migrate instead of proliferating, but in the rabbit, endothelial cells proliferate but may not provide a functional barrier [220–222]. In humans, the endothelium is thought not to proliferate at all or, if it does, not at a rate sufficient to replace lost cells since there is decreased corneal endothelial density with age. Therefore, injury to the corneal endothelium in a toxicology study is generally not an acceptable result. Human corneal endothelium has been shown in vitro to have proliferative capacity, even from aged patients, but that capacity requires a strong mitogenic signal [223]. Similar observations have been made with corneas of other species, in that there is proliferative capacity when cultured in vitro, but there is still a tendency for decreased corneal endothelial density with age for rhesus macaques, rats, mice, and rabbits. At times, some limited endothelial proliferation can be observed in laboratory animals (Fig. 5.13).
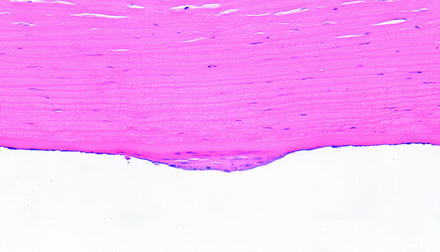
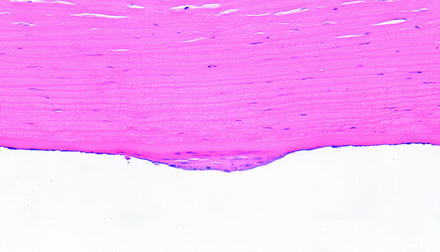
Fig. 5.13
Cornea from a dog that had a device implanted in the anterior chamber. The posterior surface of the cornea has a focal area of endothelial hyperplasia. H&E. 20× objective
With the limited regenerative capacity of the corneal endothelium, the loss of corneal endothelium is repaired by spreading and decreased density of the existing endothelium (Fig. 5.14). This can manifest as attenuation microscopically, where corneal endothelial nuclei are fewer and spaced farther apart. Detection of such a change requires detailed comparison with concurrent controls and consistent orientation of the eye, since there are differences in endothelial density dorsally as compared to ventrally. However, this is probably better assessed with specular microscopy, described elsewhere in this book. With a greater degree of endothelial loss, where the remaining endothelium cannot migrate and spread over the defect, a fibrous membrane (i.e., retrocorneal membrane) may form [224] (Fig. 5.15). The origin of the retrocorneal membrane is thought to be from keratocytes or from corneal endothelial cells that have undergone a metaplastic change to fibrocytes [225]. A retrocorneal membrane would be expected to result in permanent corneal opacity in affected areas. Loss of endothelium may also result in adherence of the iris to the posterior corneal surface which is anterior synechia.
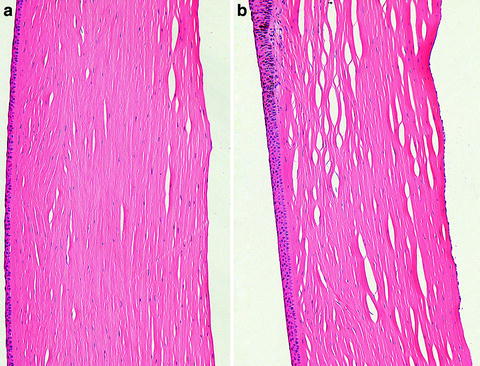
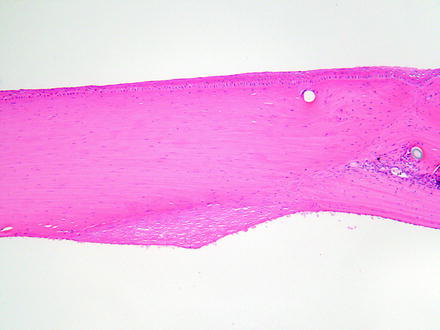
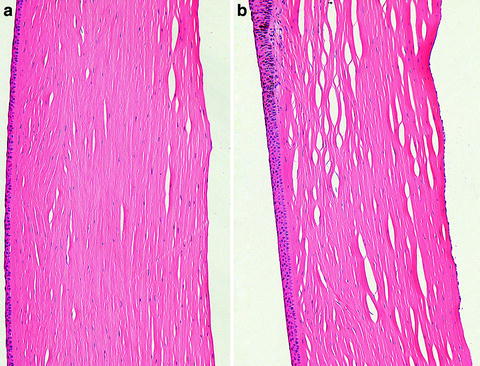
Fig. 5.14
Cornea from a monkey that had a device implanted in the anterior chamber. The inferior cornea (a) has a paucity of endothelial cells as compared to the superior cornea (b). The corneal endothelium is attenuated and widely spaced. H&E. 20× objective
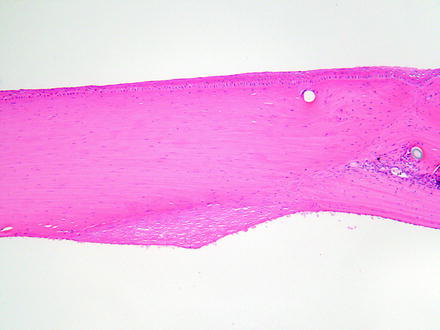
Fig. 5.15
Cornea from a rabbit 2 weeks after a surgical procedure. A retrocorneal membrane has formed at the incision site on the posterior surface of the cornea where the endothelium was lost. Note the disorganization of the stroma at the incision site and the cross sections of suture material. H&E. 10× objective
Injury to endothelial cells can be recognized ultrastructurally as loss of surface microvilli, alterations in surface apical processes, rounding of cells, separation of cells, death, and loss of cells from the basement membrane. In response to cellular stress, endothelial cells produce excess basement membrane that accumulates between the basal surface of the endothelial cells and Descemet’s membrane. This may progress to the above-mentioned fibrous metaplasia and the formation of a retrocorneal membrane [17]. Protective mechanisms associated with glutathione are important in maintaining cell viability. Endothelial cells may be damaged directly by instillation of material into the anterior chamber during surgical procedures [17].
Chemical features in the local environment including ionic concentration, bicarbonate levels, pH, and tonicity affect the corneal endothelium. Damage to endothelial cells can generally be categorized into two major mechanisms: alterations in passive permeability or alterations in function. For example, benzalkonium chloride, a surfactant, will cause endothelial cell separation and therefore will increase permeability. Dimethyl sulfoxide has been associated with corneal opacification, and this may also reasonably be related to increased permeability of the endothelial layer. Hydrogen peroxide and other drugs or substances that inhibit catalase or glutathione activity will affect endothelial membranes and ion pumps due to the increases in free radicals. Drugs that affect the endothelium can reach the anterior chamber by direct diffusion if given topically or through filtration during the formation of aqueous fluid if given systemically. Damage to the endothelium can also be a secondary event to the administration of phototoxic chemicals such as rose bengal or chlorpromazine, which produce free radicals when exposed to ultraviolet or visible spectrum light [226–228]. Anything that may affect corneal endothelial metabolism and the function of sodium-potassium ATPase pumps may result in corneal swelling.
< div class='tao-gold-member'>
Only gold members can continue reading. Log In or Register a > to continue
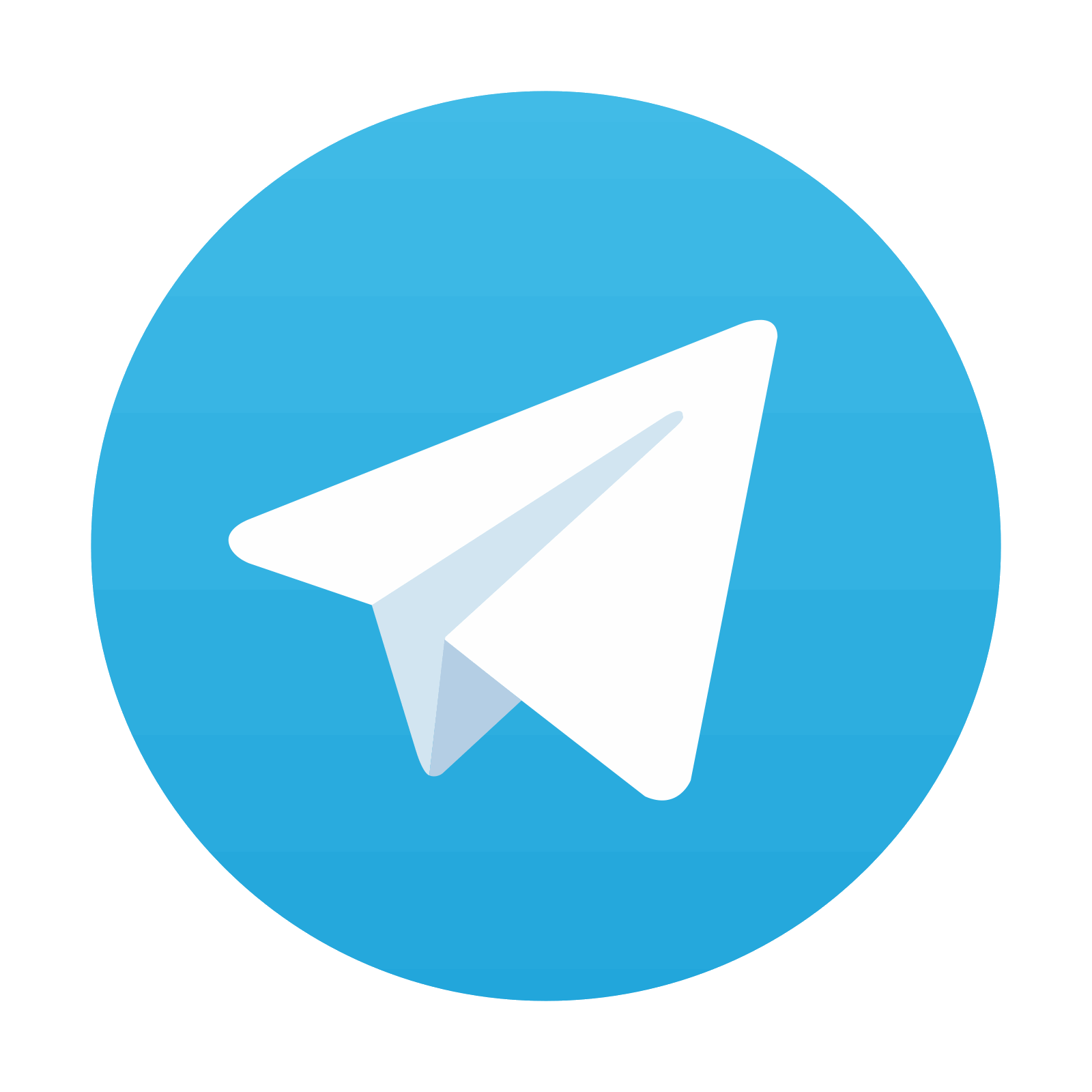
Stay updated, free articles. Join our Telegram channel
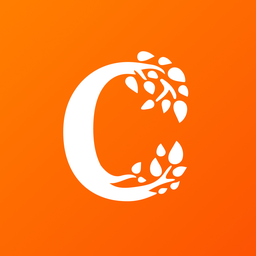
Full access? Get Clinical Tree
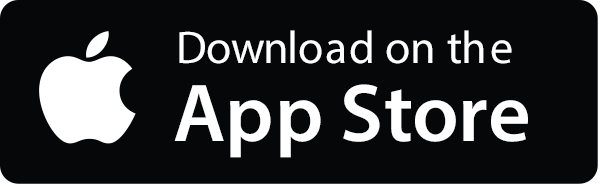
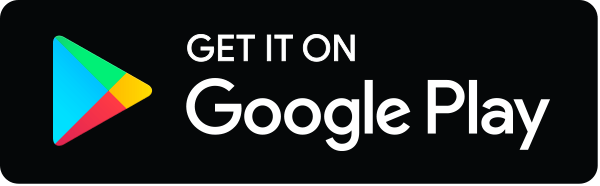