Fig. 6.1
Lens from a monkey with spontaneously occurring cataracts. Histologic changes in the lens include swelling of lens fibers (bladder cells), vacuolation, and proliferation of lens epithelium (upper right). H&E. 10× objective
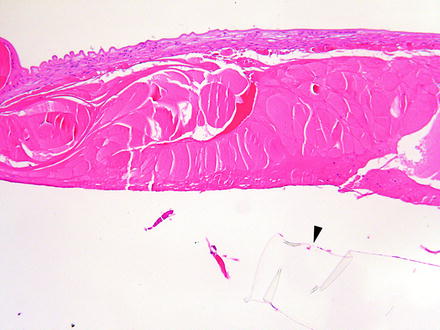
Fig. 6.2
Lens from a rabbit 2 weeks after a lensectomy procedure. Portions of the transparent implanted intraocular lens are visible (arrowhead). The anterior lenticular epithelium is hyperplastic and undergoing fibrous metaplasia. Lens fibers that were not removed during the procedure are swollen. H&E. 10× objective
6.2.3 Spontaneous Lenticular Opacities
It is common for lenticular opacities to occur in rodents [21]. Mice appear to develop more lenticular opacities than rats, and minor opacities are common in young mice [22]. Diffuse opacification and vacuoles around suture lines occur with increasing age of mice, and lenticular opacities may be strain specific (e.g., Crl:CD1®(ICR)BR mice) [22].
Various types of optical changes are considered to be background changes in the lens of rats, and some may be inherited [23]. Spontaneous changes in the lens may involve the cortex with loss of transparency or be associated with lens capsule rupture [21, 24]. Changes associated with anterior suture lines are common in older rats [20, 25, 26], and opacities involving the anterior suture lines appear to be common in spontaneously hypertensive rats [27]. Lesions of the posterior cortex may occur in the rat, especially young rats that have focal opacities associated with remnants of hyaloid vessels [20, 21]. Posterior capsular cataract appears as one or more small granular plaques and is common in older animals [21]. Swollen lens fibers are observed in a peripheral arcuate pattern in Sherman rats and as striations in the anterior cortex in Sprague-Dawley rats [20, 26, 28].
Other laboratory animals may develop spontaneous lenticular opacities. Hamsters have few spontaneous opacities, but spontaneous opacities of anterior cortex and nucleus occur in young hamsters, and striations within the cortex and posterior cortical cataract occurs with advancing age in hamsters [21]. Congenital cataracts are reported in the guinea pig [29]. Posterior cortical pinpoint lens opacities have been described in the Göttingen minipig and Yucatan micropig [30].
In Beagles, anterior and posterior suture opacities have been described in animals at approximately 15 weeks to 3 years[31–36]. Bilateral vacuoles or punctate opacities near suture lines at the periphery of the posterior cortex occur in young laboratory beagles, and age-related lenticular changes are well characterized in laboratory beagle dogs [21]. Posterior sutures may be pronounced in very young dogs [31, 32] and in older dogs [21, 33]. Cataracts that occur in young beagles often involve the posterior aspect of the lens and may be pinpoint [21, 34]. Findings include posterior polar subcapsular streaks or small plaques [35]. Posterior capsular and cortical opacities increase with age in the beagle dog [21, 33, 36].
With the continuous production of lens fibers, there is constant compression of the lenticular nucleus, and the optical density of the nucleus increases slowly with age (i.e., nuclear sclerosis) [20, 26]. Nuclear sclerosis must be differentiated from nuclear cataract. Nuclear cataract can occur, especially in rodents, and is more common in mice than rats. Spontaneous nuclear cataracts are rare in rats but do occur in Sprague-Dawley rats. Unilateral nuclear cataract has been reported as age-related in Sprague-Dawley rats. Inherited nuclear cataracts also occur in Sprague-Dawley rats [21, 27, 37]. Nuclear opacities occur in spontaneous hypertensive rats of the Okomoto strain, and nuclear cataract has been observed in hamsters [21]. A nonprogressive focal opacity of the fetal nucleus has been reported in beagles, but focal opacities and spontaneous nuclear cataracts are rare [25]. Focal nuclear opacities occur in Göttingen minipig [38], dog, Sprague-Dawley rat, and Yucatan micropig. Increases in nuclear densities after 1 year have been reported in the rat [25].
Granulomatous inflammation focused on the lens occurs in rabbits, especially dwarf rabbits, and occurs with rupture of the lens capsule (i.e., phacoclastic uveitis) [39]. Although not always detected in the affected globe, Encephalitozoon cuniculi is thought to be the cause. Although the pathogenesis is not completely understood, vertical transmission occurring during lens development is a possible source of the infection.
Aging associated with cataract formation has been reviewed [40] and is thought to be the net result of oxidative stress [24]. Oxidative stress decreases superoxide dismutase and GSH activities, increases free radical formation, and increases protein aggregation [40–42]. The Emory mouse is a model for age-related cataract in humans.
6.2.4 Lenticular Toxicity
Only a few substances have been shown to cause cataract in humans, but many compounds have been shown to cause cataract in animals [18, 43–45]. This may be due to the large number of compounds tested in animals. Cataract may be caused by many different mechanisms and may be induced in laboratory animals by various factors [18, 46, 47]. Factors influencing cataract formation include aging, disrupted metabolism, nutritional deficiency, and exposure to oxygen radicals, X-ray radiation, microwave radiation, gamma radiation, and UVA or UVB light [18, 46, 48, 49].
“Sugar” cataract is produced by high concentrations of galactose, xylose, or glucose and has been demonstrated in rats [18, 48]. These sugars are converted to sugar alcohols which become trapped in the lens and osmotically attract water into the lens. This results in excessive hydration, swelling of cells and fibers, and fluid between fibers. High lens glucose is metabolized by aldose reductase pathway to sorbitol, and increased amounts of sorbitol causes hyperosmotic stress on lens fibers with the potential for cataract. The high blood glucose conditions of diabetes mellitus or induced by administration of alloxan or streptozotocin cause persistent hyperglycemia and eventually a “sugar” cataract in rats and rhesus monkeys [48, 50]. Polyol (sugar alcohols such as sorbitol and xylitol) accumulation and protein glycation in diabetic mice have been described as well [41]. In addition to increased sugars, tryptophan deficiency can cause cataract in the rat [51].
Several compounds cause cataract. Acetaminophen causes cataract by decreasing GSH levels [18]. Naphthalene causes cataract through a P450-glutathione-dependent mechanism [52] and is cataractogenic and retinotoxic (retinal edema) in the rabbit [53]. Diquat induces the production of free radicals and structural changes in lens fibers in the rat and dog [50]. Repeated subcutaneous injections of buthionine sulfoximine causes osmotic swelling along suture boundaries in the anterior cortex but eventually spreads to the rest of the lens [24]. Administration of phenylalanine or chlorophenylalanine results in a protein disturbance and cataract [50]. Disruption of lipid metabolism may result in cataract. Triparanol inhibits cholesterol synthesis and thus cell membrane production [48]. Administration of aminopyridine inhibits oxidosqualene cyclase and blocks cholesterol synthesis at hydroxymethylglutaryl-coenzyme A (HMGCoA). The result is necrosis and swelling of fibers at equator [50]. U18666A, an inhibitor of enzymatic reduction of desmosterol to cholesterol, produces permanent nuclear cataract. Agents that cause hypolipemia, such as styryl-hexahydroindolinol and AY-9944, have been associated with cataracts [54].
Cataract formation may have multiple causes. Selenite causes nuclear cataract in young rats [55]. Inhibition of enzymes or disruption in protein metabolism causes cataract and may be due to compounds such as chlorophenylalanine, phenylhydrazopropionitrile, mimosine, and naphthalene [18]. Administration of naphthoquinone inhibits Na/K-ATPase leading to an electrolyte disturbance and osmotic swelling of lens fibers [50]. Radiomimetic cataracts are similar to those produced by radiation [18]. Compounds that produce this effect include busulfan, dibromomannitol, dimethylaminostyrylquinoline, iodoacetate, nitrogen mustard, tretamine, and triaziquone. Posterior subcapsular cataract is produced by a variety of glucocorticoids in humans, but these are difficult to replicate in animals [18]. Uncoupling of oxidative phosphorylation caused by 2,4-dinitrophenol produces cataract in humans, but not in the dog and rat [50].
6.2.5 Lenticular Epithelial Alterations
Changes in uniformity, such as swelling of lens fibers and variation in shapes of lens cells, may be noted in the germative zone before opacity occurs. Lenticular epithelial cells may undergo focal hyperplasia in older rats. Administration of 4-diethylaminoethoxy-α-ethyl-benzhydrol to rats caused inflammation of the cornea and anterior uvea with secondary lenticular epithelial proliferation followed by pyknosis and fiber degeneration. Perturbation of cell division may occur following ionizing radiation or the administration of 4-p-dimethylaminostyryl and busulfan [50]. UV light is a possible factor in age-related cataracts because UVB radiation causes epithelium to undergo apoptosis and hyperplasia, as well as anterior suture disintegration. Vacuolation of lens epithelium occurs following administration of SK&F 86466, an alpha2 adrenoreceptor antagonist. Antineoplastic antimitotic agents affect mitotically active cells in the germative zone of lens epithelium with changes noted at the equator [18]. Lenticular epithelial cells may undergo focal proliferation in the aged rat or following surgery or trauma (Fig. 6.2).
Neoplasms of the lens do not appear to occur spontaneously in laboratory animals.
6.2.6 Lens Capsule Alterations
The lens capsule is continuously produced by the anterior lens epithelium, such that the anterior capsule will increase in thickness with age and may be notably thicker than the posterior capsule. With intraocular surgical techniques in preclinical studies, there is the potential for nicks in the lens capsule, which may result in adjacent opacities and lens fiber changes. Infrequently, intravitreal injections of some soluble therapeutics may diffuse into the lens capsule and produce abnormal staining, although producing no ophthalmologic changes. One example is basophilic staining of the lens capsule and optic disc following intravitreal injection of oligonucleotides (Figs. 6.3 and 6.4).
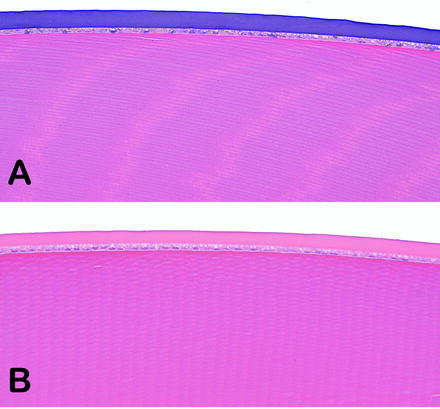
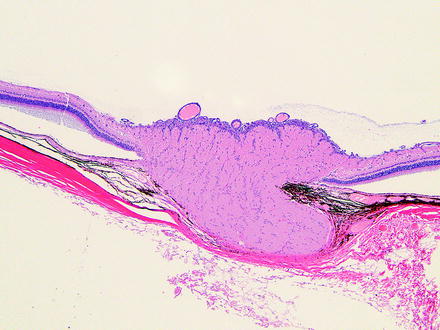
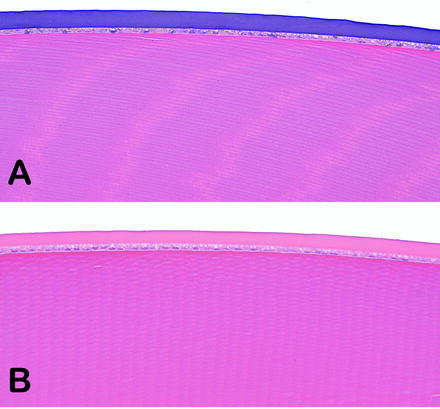
Fig. 6.3
Lenses from rabbits on an intravitreal injection study. (a) The soluble test article tended to accumulate in the lens capsule and caused basophilic staining. (b) Lens capsule of a vehicle-treated rabbit was unremarkable. No abnormal ophthalmic examinations findings were observed. H&E. 20× objective
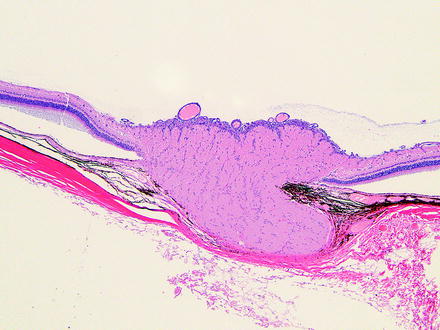
Fig. 6.4
The optic disc from the same rabbit in Fig. 6.3a. The inner limiting membrane was basophilic. No funduscopic examination correlates were observed. H&E. 5× objective
6.3 Vitreous Body
The vitreous humor is a transparent, jellylike material that fills the vitreous chamber [3, 5, 13, 56]. Alterations of the vitreous may be congenital, spontaneous, or iatrogenic associated with drug administration.
6.3.1 Spontaneous Vitreal Alterations
Many of the spontaneous background findings involving the vitreous body are various types of remnants of embryological structures, especially the hyaloid vessels. The embryologic vessels surrounding the developing lens (the tunica vasculosa lentis), hyaloid vessels, or both may persist. If there is a proliferation of connective tissue in addition to the blood vessels, the finding is classified as persistent hyperplastic primary vitreous (PHPV). This finding has been reported in the Sprague-Dawley rat [57]. Persistent hyaloid vessels have been reported in rats [20, 25, 57–60], Swiss mice [22], Göttingen minipigs [38], and Yucatan micropigs [61] but is a common congenital finding in many species. Persistent hyaloid vessels are rare in laboratory primates [53]. In the rat, there are patent hyaloid arteries that persist up to 6 days of age and close between 1 and 3 weeks [20]. Remnants of the hyaloid vessels are still present at 6 weeks in a majority (60%) of rats with a few (2%) containing blood. By the age of 26 weeks, a few (5–6%) of the rats have hyaloid remnants and an occasional rat (1%) will have a hyaloid remnant at 1 year of age.
Intravitreal hemorrhage is rare in dogs but common in rats [53]. Vascularization of the vitreous may cause intravitreal bleeding which may lead to vitreal traction bands on the retina. When hyaloid vessels are patent, blood extends into the vitreous. This is the main cause of vitreous hemorrhage reported in the mouse [Crl:CD1(ICR)BR] [22] and rat [20, 57, 58]. Erythrocytes are engulfed by phagocytic cells resulting in brown-pigmented cells in the vitreous [57]. Persistent hyperplastic vitreous causes defects in the posterior lens capsule which may result in a posterior polar cataract [53]. Preretinal arteriolar loops which extend into the vitreous are uncommon findings in beagles and can be detected during the pretest study period. They are caused by a defect in the inner limiting membrane of the retina [53].
In humans, rheological (the gel-liquid state of the vitreous) changes may occur in the central vitreous. A liquefaction process may occur in dogs and may cause separation of the posterior cortex from the retinal inner limiting membrane, predisposing to retinal tears and rhegmatogenous retinal detachment [5]. Hyaluronic acid provides viscoelasticity but also acts as a barrier to the diffusion of macromolecules into the vitreous, unless that barrier is disrupted by trauma. The vitreous is a storage site for retinal metabolites and protects the lens and retina from toxic compounds. Pathologic processes leading to decreased hyaluronic acid concentration and vitreal liquefaction will affect the nutrient supply, waste removal, and drug delivery in the posterior segment. In most species, holes and liquefaction (i.e., syneresis) occur in older individuals, especially in the central or intermediate zones [5].
6.3.2 Iatrogenic Vitreal Findings
Most of the changes in the vitreous in ophthalmic studies are iatrogenic and due to the administration of a compound or device to treat the retina [63]. These findings include inflammation, plasmoid vitreous, hemorrhage, liquefaction, and displacement (possibly causing retinal detachment). Inflammatory infiltrates often consist of macrophages, which will phagocytose and attempt to clear poorly soluble materials via migration out the optic nerve. Inflammatory infiltrates and hemorrhage may lead to progressive neovascularization [21]. The vitreous may prolapse through intravitreal injection tracks.
6.3.3 Toxicologic Vitreal Findings
In addition to the physical changes associated with the intravitreal administration of a compound, there may be a toxic effect involving the vitreous and the adjacent structures (i.e., the lens and retina). Intravitreal injection of commercially available ketorolac tromethamine in rabbits resulted in retinal toxicity [64].
6.4 Retina
There are many references covering the embryology, anatomy, physiology, and biochemistry of the retina, and retinal anatomy is also covered elsewhere in this book [3, 5, 13, 65]. In general, the retinal structure is similar among species, but there are a few differences in ocular anatomy among laboratory animal species, for example, the macula in primates [5, 66]. The retina consists of the inner transparent sensory (neurosensory) retina and the outer retinal pigmented epithelium (RPE) separated by a potential space, the subretinal space. Axons of retinal ganglion cells converge to form the optic nerve. The peripheral sensory retina abruptly becomes the inner ciliary epithelium and the RPE abruptly becomes the pigmented ciliary epithelium at the ora ciliaris (ora serrata in humans and nonhuman primates).
The layers of the retina, from external to internal, are the RPE, the photoreceptor layer (i.e., layer of rods and cones), the outer limiting membrane (OLM), the outer nuclear layer (ONL), the outer plexiform layer (OPL), the inner nuclear layer (INL), the inner plexiform layer (IPL), the ganglion cell layer (GCL), the nerve fiber layer (NFL), and the inner limiting membrane (ILM).
Generally, morphologic changes in the retina are permanent, so early detection of toxicity is important [67]. The primary methods of clinically evaluating the retina for toxicity are indirect ophthalmoscopy (fundoscopy) and flash electroretinogram (ERG), although clinical evaluation may also involve fluorescein angiography, confocal scanning laser tomography, ultrasonography, and optical coherence tomography [2, 53, 66, 68, 69].
Terms used to describe changes in the retina should be descriptive and accurate. For example, the outer displacement of a photoreceptor nucleus into the layer of inner and outer segments should be referred to as photoreceptor displaced nuclei which may be normal or may be one of several changes that may cumulatively be referred to as retinal degeneration. Since the retina is composed of many different types of cells, the use of retinal degeneration may be a vague term. For one study, retinal degeneration might represent loss of ganglion cells, and in another study, retinal degeneration might represent changes associated with photoreceptors. Use of precise diagnostic terms for retinal findings helps to eliminate confusion.
6.4.1 Spontaneous Retinal Alterations
Retinal findings generally involve retinal vessels, photoreceptors, or ganglion cells. Spontaneous findings need to be differentiated from treatment-related toxicities. Vascular findings are often a reflection of a systemic condition such as spontaneous preretinal arteriolar loops in dogs or possibly alterations associated with atherosclerosis such as calcification or changes associated with hypertension leading to hemorrhage or edema. Occasionally, spontaneous retinal changes are observed without a clear pathogenesis (Fig. 6.5).
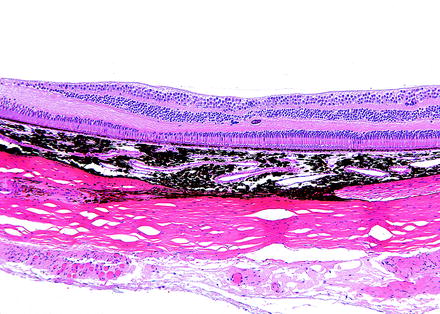
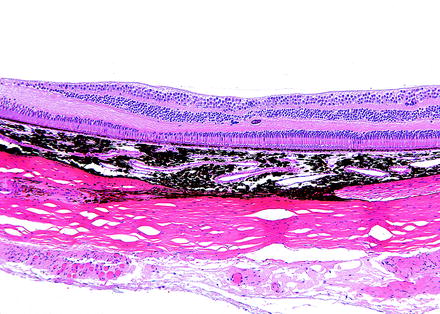
Fig. 6.5
Retina from a monkey. Fovea is to the left. On prestudy ophthalmic examination, a retinal “scar” was identified adjacent to the fovea. The retina has loss of cells in the outer nuclear layer and inner nuclear layer. The photoreceptor, inner plexiform, and outer plexiform layers are focally thinned. The outer plexiform layer has a focus of mineralization. H&E. 10× objective
Alterations involving photoreceptors include dysplasia, dystrophy, and degeneration. The term retinal dysplasia is used to describe focal or multifocal disorganization of the sensory retina due to faulty development and is usually characterized by retinal rosettes, abnormal alignment of photoreceptor cells, and possibly cellular degeneration [70]. Retinal folds are occasionally diagnosed as retinal dysplasia clinically and microscopically, but should be given a separate diagnosis. Since the retina of the rat at birth is considered to be equivalent in development to the human retina at 4–5 months gestation, it is possible to create experimental developmental findings in the eyes of rat pups [70]. For example, retinal dysplasia has been produced by administration of cytosine arabinose, cycasin, N-methyl-N-nitrosourea (NMU), and trimethyltin [37, 70–72]. Spontaneous retinal dysplasia occurs in many laboratory animals, especially rats and rabbits, but does occur rarely in laboratory beagles [53]. In the rat, retinal dysplasia generally consists of unilateral linear elevations of the retina of varied lengths [37]. Sprague-Dawley rats have different types of retinal dysplasia. Retinal dysplasia may be unilateral or bilateral and characterized by focal or occasionally diffuse absence of outer layers of the sensory retina. This form occurs more frequently in males and increases in incidence with age [73]. Another form of retinal dysplasia occurs in Sprague-Dawley rats at 7–10 weeks of age and is referred to as linear retinopathy, retinochoroidal degeneration or atrophy, retinal dysplasia or dystrophy, or choroid defect [21, 25, 37, 57, 73, 74]. The finding is generally unilateral and consists of thinning from focal loss of the outer layers of the sensory retina with direct abutment of the INL to the choroid or even sclera.
Inherited retinal degeneration is a condition that generally affects photoreceptors, the RPE, or both and varies in the time of onset with a tendency to progress in severity. The hereditary condition occurs in several strains of mice, including several mutant and transgenic mouse strains, rats, and monkeys, but is rarely observed in hamsters and laboratory beagles [21, 22, 53, 75–80, 81–85]. One example is retinal dystrophy in the Royal College of Surgeons (RCS) rat that has a spontaneous mutation for a gene that encodes a receptor critical for phagocytosis of shed outer segments [80, 84, 85]. The RCS rat lacks the ability to phagocytose shed discs, resulting in photoreceptor loss, reduced numbers of nuclei in the ONL, and debris between the RPE and photoreceptors [77]. Other examples of retinal dystrophy include Wag/Rij and Osborne-Mendel stains of rats [53, 78, 84].
Treatment-related degeneration of photoreceptors needs to be differentiated from spontaneous (including inherited) retinal degeneration, especially senile retinal degeneration, a condition characterized by the gradual decrease in the number of photoreceptors, especially rods, and thinning of the ONL with age for all of the laboratory animal species, especially rodents [66]. Since rodents have a relatively short lifespan in comparison to other laboratory animals, it is easier to observe senile retinal degeneration in these species. Senile retinal degeneration can affect enough of the retina such that retinal atrophy is the preferred term. Changes can advance to a degree that the retina consists of just a fibrous layer with glial cells and occasional neurons [21, 24, 74, 86–92]. Age-related effects have been reported in various laboratory animals including aged CD-1 and B6C3F1 mice, and in albino rats including Chbb/THOM strain, Fischer 344, Sprague-Dawley, and Wistar-Furth rats [21, 74, 86–92]. For example, the ONL in F344 rats decreases in cellular thickness from 12 cells thick at 3 months to less than 8 cells thick at 18 months [90]. In Wistar rats, the incidence of senile retinal atrophy can reach 10% in both males and females at 2 years [88].
Since the peripheral retina is thinner than the central retina, degeneration associated with aging is more noticeable in this region and may begin with microcystoid changes in the RPE. Changes typical of senile peripheral retinal degeneration include retinal thinning with a loss of nuclei in the ONL and INL, fusion of the nuclear layers, displacement of photoreceptor nuclei into the inner and outer segment layers, hypertrophy of the RPE, and possibly migration of RPE cells or macrophages into the sensory retina [21, 53, 79, 87, 90, 93]. Infiltration of heavily pigmented cells into the subretinal space may be detached RPE cells or possibly macrophages.
Some strains of mice, especially those background strains used for knockin/knockout transgenic studies such as FVB mice, carry a photoreceptor gene mutation (rd/rd) that leads to progressive retinal atrophy with age [75, 82] (Fig. 6.6). Selection of an appropriate strain is important if the eye is to be a focus of interest in these engineered animal models [94].
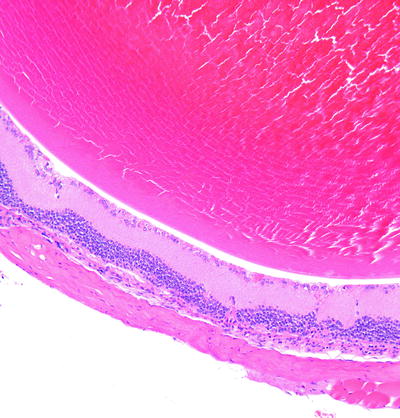
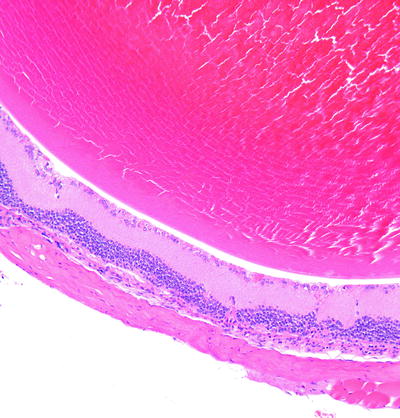
Fig. 6.6
Eye from an FVB mouse. The retina has loss of the photoreceptor and outer nuclear layers due to a mutation in a photoreceptor gene. H&E. 10× objective
The normal, gradual loss of photoreceptors with age may be detected by the displacement of photoreceptor nuclei into the inner and outer segment layers in normal retinas of humans and laboratory animals including monkeys, pigs, cats, dogs, rabbits, guinea pigs, rats, mice, and hamsters [93, 95]. Displaced photoreceptor nuclei (PDN) are generally low in number and contain a normal density of chromatin, but the number may vary. Occasionally, nuclei are small with condensed chromatin and referred to as pyknotic nuclei (especially in nonhuman primates). These PDN are distributed over the entire retina but more frequently in the periphery. They have a tendency to be more frequent in the retina of very young rats, rats exposed to high ambient light intensities, aged rats, or in globes with ocular disease (e.g., senile macular degeneration or cataract), or in the retina of individuals with systemic disorders (e.g., diabetes mellitus and septicemia). The nuclei eventually undergo degeneration and are removed by the RPE. Since PDN may or may not be occurring with other findings in the retina, PDN may be a separate diagnosis.
A condition to consider when evaluating the retina of albino animals for toxicity, especially from long-term studies, is phototoxic or light-induced retinopathy. The exact mechanism of light-induced retinal degeneration is unknown but may involve phototransduction or oxidative mechanisms with free radical liberation in the photoreceptor outer segments [96]. The primary ocular mechanism for controlling light exposure is constriction of the pupil by the pigmented iris [66, 97, 98]. Light-induced retinal degeneration has been reported in pigmented rats when pupils are dilated and in pigs exposed to constant illumination for at least 1 month when their pupil size remained relatively large with constant illumination [97, 99].
Uveal pigmentation of nonalbino animals, especially of the iris, absorbs light and protects the retina from light-induced damage [100–104]. This condition has been described in rats, mice, monkeys, rabbits, and miniature pigs [22, 46, 57, 99, 100, 104–108]. In rats, females have a tendency to be more affected than males. Exposure to light contributes to the natural aging process, but short-exposure to high light intensity or long exposure to low levels of artificial lighting may cause photoreceptor degeneration [87]. For example, photoreceptor degeneration is more pronounced in rats housed under light intensity of 32 foot-candles than rats housed under light intensity of 1 foot-candle, but even though the overall room light intensity may be within the acceptable limits, the light exposure of animals on the top of a cage rack has greater light exposure than animals on the bottom of the cage rack [87, 88, 90, 100, 103, 109, 110]. In long-term toxicology studies, age-related and light-associated retinal lesions are common. Therefore, it is critical that a light intensity (12 h light/12 h dark) of 60–200 lux not be exceeded [66, 87, 90, 111, 112]. In addition to light intensity, other factors that influence the development of phototoxic retinopathy include wavelength, duration of exposure, length of time for dark-adaptation, age of initial exposure, maturity of the retina, body temperature, albinism, decreased prior daily light exposure, changes in light/dark cycle length, and diet including a deficiency or excess of vitamin A or deficiency of vitamin E or taurine [21, 46, 53, 66, 91, 100, 101, 113, 114]. In general, light-induced retinal degeneration is more prominent in the central retina, and rods are more sensitive than cones. Alterations include a decrease in the thickness of the ONL; disorganization and thinning of the layers of outer segments and inner segments; the presence of normal appearing or darkly stained, pyknotic photoreceptor nuclei in the layers of the outer and inner segments and in the subretinal space; and possibly astrocyte proliferation and Müller cell activation [100, 112, 115]. Normally, the ONL is thicker than the INL, but with light-induced retinopathy, the thickness of the ONL may appear similar to the thickness of the INL, and the layer of inner and outer segments will appear thinner and disorganized. The ONL gradually thins and eventually disappears.
A common change occurring especially in dogs, monkeys, and humans is peripheral cystoid retinal degeneration (Fig. 6.7) [39, 53]. It is described as a normal change in virtually all human patients by 8 years of age and consists of single to multiple vacuoles in the peripheral retina in the INL, IPL, and OPL [116]. This finding is noted in the superior nasal quadrant of dogs as early as 8 weeks and in the peripheral temporal retina of monkeys with age. This background change should be distinguished from retinoschisis, which is splitting of the retinal layers [39, 53].
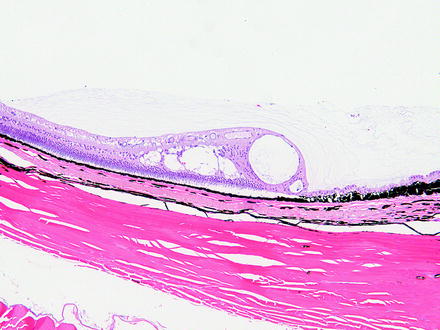
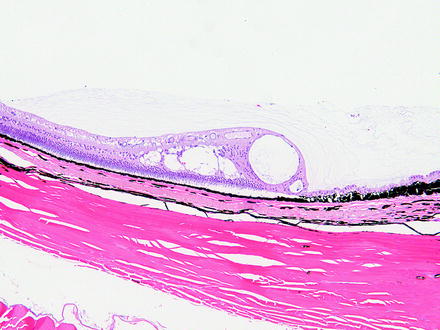
Fig. 6.7
Peripheral retina from a dog with peripheral cystoid retinal degeneration. Multiple cyst-like spaces expand the retina with loss of layers and organization. H&E. 10× objective
Ganglion cells are lost as a spontaneous condition in rhesus and cynomolgus monkeys as part of idiopathic optic neuropathy. Loss of ganglion cells in the macular region correlates with loss of axons in the temporal aspect of the optic nerve [117, 118].
The sensory retina may focally bulge inward with the photoreceptors possibly separating from the underlying RPE forming a retinal fold [37]. Spontaneous retinal folds have been reported in many species of laboratory animals including mice, rats, (Sprague-Dawley, Wistar), rabbits, and dogs, especially young animals [22, 53, 57]. In young animals, when present histologically, these may be Lange’s folds, which are artifactual folds related to fixation [119, 120]. Some retinal folds may be recognized during the prestudy examination and appear as short gray to brown lines in the nontapetal area of the canine fundus or short gray or white lines [53, 66]. This finding is common in young beagles and may decrease with age. Folds usually affect outer layers of the retina and, in rabbits, they occur in the region of the medullary rays [53]. They may be associated with preretinal traction membranes or subretinal hemorrhage and develop into focal retinal detachment [33, 53]. Retinal folds may be congenital in Sheffield-Wistar rats and associated with microphthalmia or other intraocular anomalies. Depending on the plane of histologic section, retinal folds may resemble a rosette [121].
Spontaneous retinal detachment is uncommon in laboratory animals but may occur rarely in B6C3F1 mice, rats (Sprague-Dawley), dogs, and laboratory monkeys [24, 53, 57]. Funduscopically, retinal detachment appears as a gray membrane and may be associated with preretinal traction membranes, rhegmatogenous change associated with retinal holes or tears, or the presence of fluid or hemorrhage within the subretinal space [53]. Traction membranes may develop secondarily to disruptions of the vitreous body and be due to contraction bands of organized tissue within the vitreous or be due to neovascular disease or intraocular surgery. Serous or exudative retinal detachment consists of fluid in the subretinal space and may develop secondarily to hypertension. Other causes include retinal detachment secondary to buphthalmos, caused by glaucoma, trauma, and the administration of compounds. Dithizone causes retinal edema and exudation, leading to secondary retinal detachment, and administration of diphenyl thiocarbazone causes retinal detachment in the dog, but not in cat, rat, rabbit, or monkey [18, 53].
True retinal detachment needs to be differentiated from artifactual retinal detachment. In addition to obvious causes, such as traction membranes and the presence of fluid or hemorrhage in the subretinal space, the RPE cells are hypertrophied and individualized, giving them a “tombstone” appearance when the retinal detachment is not artifactual. With time, retinal detachment will result in photoreceptor degeneration.
6.4.2 Retinal Inflammation, Trauma, and Gliosis
Retinal inflammation may occur spontaneously or may be induced experimentally. Spontaneous inflammation of the retina is rare in laboratory animals but may occur as a unilateral finding secondary to trauma [53, 122]. Retinal inflammation usually occurs secondarily to inflammation of the choroid and results in retinal degeneration. For example, multifocal serous chorioretinitis has been reported in the beagle dog with focal retinal detachment and focal loss of photoreceptors. Experimentally in mice, there are virally induced retinitis models and autoimmune disease models induced by immunization with retinal S-antigen or interphotoreceptor retinoid-binding protein [24]. The latter model involves an infiltration of neutrophils and mononuclear inflammatory cells in the retina.
Retinal gliosis has been observed as a focal finding localized around the optic disc and is confined entirely to the retina in rats (Fig. 6.8) [123]. The finding consists of an expanded NFL containing cells with elongated nuclei, fibrillar eosinophilic cytoplasm, and indistinct membranes. These cells are positive for anti-glial fibrillary acidic protein (GFAP) indicating their origin as glial cells [123].
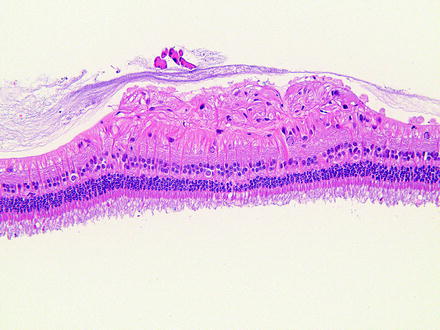
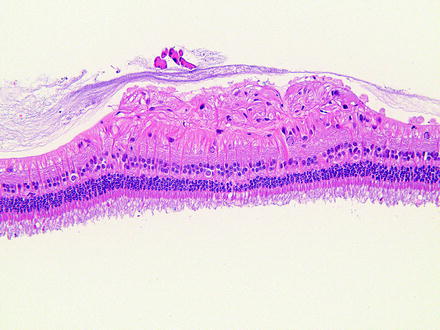
Fig. 6.8
Rabbit retina with focal gliosis expanding the nerve fiber layer. Retinal gliosis is infrequent and of unknown origin. H&E. 20× objective
Focal retinal degeneration can occur as a consequence of surgical trauma from procedures such as phacoemulsification lensectomy (Fig. 6.9).
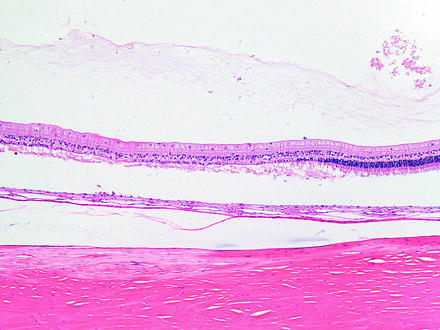
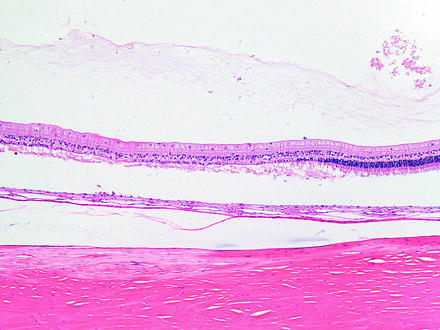
Fig. 6.9
Retinal degeneration in a rabbit 2 weeks after phacoemulsification lensectomy using hydrodissection. There is loss of photoreceptors which is thought to be associated in the surgical procedure. H&E. 10× objective
6.4.3 Retinal Pigment Epithelium Alterations
Changes in the RPE have been reviewed [124, 125]. Because of the functional relationship between the RPE and photoreceptor cells, photoreceptor changes are frequently secondary to RPE changes. Toxicity-related alterations involving the RPE must be differentiated from artifacts. For example, the presence of crystalloid bodies between RPE and tapetum lucidum is an artifact observed in control beagles considered to be associated with glutaraldehyde fixation.
There are several morphologic changes that can be observed in the RPE. One common change is hypertrophy. Hypertrophy may be diffuse and uniform, possibly due to an increase in smooth endoplasmic reticulum from increased metabolic activity of the cells or focal with or without an apparent cause. Focal hypertrophy of RPE cells is often associated with retinal detachment (Fig. 6.10), retinal folds, or trauma. The affected RPE cells appear individualized with a domed apical portion (“tombstone” appearance). Additional changes in the RPE include retraction of apical pigment granules, loss of polarity, hyperplasia, migration into the subretinal space or sensory retina, accumulation of deposits or cellular components, degeneration, fibroblastic or osteoblastic metaplasia, and the formation of subretinal membranes.
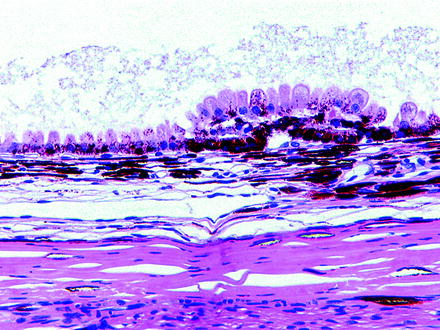
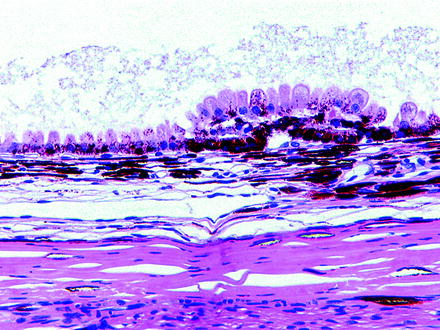
Fig. 6.10
Retinal pigment epithelium from a control dog on a 3-month oral toxicity study. The retina was noted to have tears early in the study and had completely detached by the end of the study. Cause of tears and detachment were undetermined. The underlying RPE has undergone hypertrophy and hyperplasia, demonstrating the classic “tombstoning” appearance. H&E. 20× objective
Since the RPE engulfs and degrades photoreceptor outer segments, lipofuscin may accumulate with age [125]. As this occurs, the number of melanin granules decreases. This change is especially noticed as a spontaneous finding in the RPE of rabbits, especially Dutch belted rabbits (Fig. 6.11). The brown deposits which accumulate within the cytoplasm of rabbits have the ultrastructural appearance of lipofuscin. Affected cells often occur around the optic disc or under the most peripheral retina, but may be present in other locations. Eventually, the cells extend into the subretinal space and become disassociated with outer segments. There is often concurrent focal retinal detachment with degeneration.
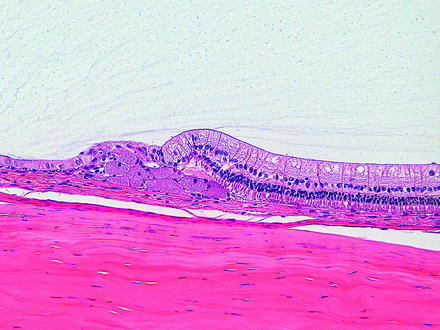
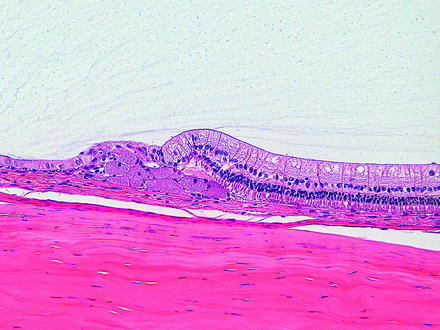
Fig. 6.11
Peripheral retina from a rabbit. There are multiple hypertrophied retinal pigment epithelial cells with granular cytoplams due to lipofuscin, occurring as a spontaneous change. H&E. 20× objective
Loss of cellular polarity, loss of apical villi, and depigmentation occur when the RPE cells are responding to injury or may be observed as a spontaneous change in the retinas of rabbits. Injured RPE cells retract melanosomes from the apical processes and become rounded, giving the appearance of hypertrophy. Rounded RPE may migrate into the subretinal space. RPE cells have a distinct morphology with apical microvilli and basal infoldings. Loss of apical microvilli may be indicative of impaired phagocytosis of photoreceptor discs, and there may be a reduced number of phagolysosomes. Depigmentation is usually from a fusion of melanosomes, and a loss of basal infoldings may be indicative of impaired transport processes.
RPE cells may undergo hyperplasia with or without metaplasia in response to stimuli that include injuries, chronic inflammation, and long-standing retinal detachment with traction. Hyperplastic RPE cells appear immature with limited differentiation and may form multiple layers between the choroid and the photoreceptor layer. RPE cells also have the potential to differentiate into fibroblasts and secrete a collagenous extracellular matrix forming a membrane between the RPE and Bruch’s membrane, which may consist of multiple cell layers interspersed between collagen-rich extracellular matrix. The membranes may be vascularized or nonvascularized.
Deposits of extracellular matrix components may accumulate between the RPE and Bruch’s membrane in monkeys [125] and other species (Fig. 6.12). These deposits are similar to drusen which occur as a degenerative change in the human eye. Drusen-like bodies consisting of subretinal concretions on Bruch’s membrane can occur in the macula of nonhuman primates as an aging change and may be associated with focal retinal detachment [66, 126, 127] (Fig. 6.13).
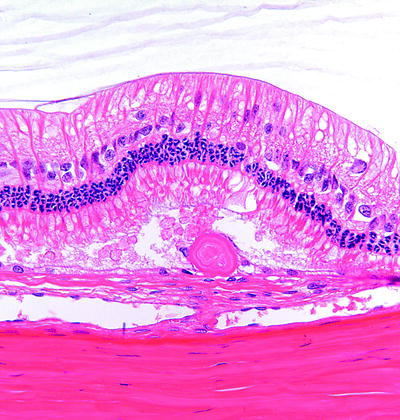
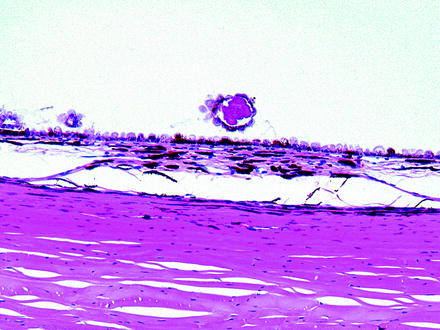
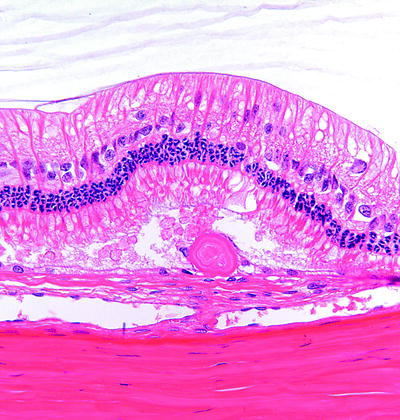
Fig. 6.12
Rabbit peripheral retina with a drusen-like deposit located below the photoreceptor layer and associated with the RPE. H&E. 40× objective
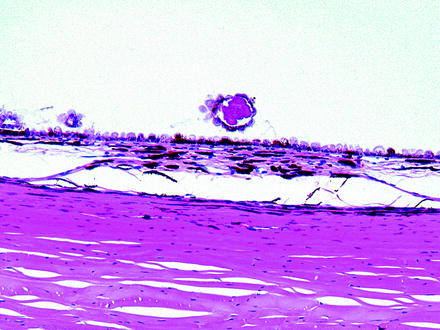
Fig. 6.13
Drusen-like deposits from the same dog as in Fig. 6.10. The drusen-like deposit is in an area of focal retinal detachment. Note that the drusen-like deposit in this animal appears to be surrounded by RPE cells. H&E. 10× objective
6.4.4 Retinal Toxicity: General
Retinal and RPE changes associated with various drug and chemical toxicities have previously been reviewed [1, 18, 44, 86, 128, 129]. Alterations involving the sensory retina consist of vascular changes, degeneration of ganglion cells, degeneration of photoreceptors, degeneration of other neurons, and reactive glial cells. Müller cells may be a target of toxicity, such as in D, L α-aminoadipic acid toxicity, with changes consisting of cytoplasmic vacuolation and necrosis [130]. Some compounds, such as trimethyltin, fenthion, and D,L-2-amino-3-phosphonopropionate, may produce alterations in more than one type of retinal cell [18, 44] (Fig. 6.14). Trimethyltin most frequently and most severely affects the inner segments of photoreceptors resulting in intracytoplasmic, membrane-bound vacuoles and dense bodies, but changes are also present in ganglion cells and neurons of the INL [131]. D,L-2-amino-3-phosphonopropionate is structurally related to aspartate, an excitatory amino acid neurotransmitter, and causes severe degeneration of all retinal layers of the neonatal rat.
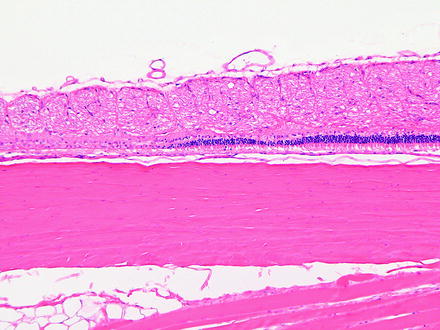
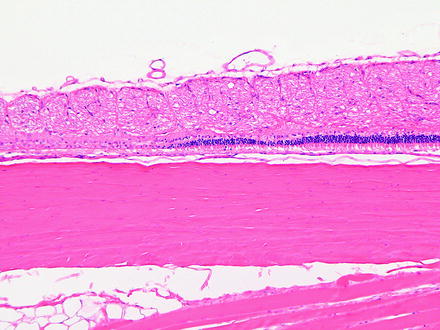
Fig. 6.14
Segmental retinal degeneration in a rabbit following an intravitreal injection. H&E. 10× objective
The predicative value of retinal findings in laboratory animals with respect to the determination of ocular toxicity may vary between rodent and nonrodent species and between animals and humans [132]. For example, enrofloxacin is an antimicrobial agent that has interspecies differences in sensitivity to retinal toxicity [133, 134]. Changes include acute blindness, ophthalmoscopic evidence of increased tapetal reflectivity, attenuation and loss of retinal blood vessels, and changes in tapetal coloration [133, 134].
Retinal lesions in animals may be difficult to correlate to retinal lesions in humans. Some drugs (e.g., ethambutol) that cause retinal lesions in laboratory animals do not appear to cause visual disturbances or retinal changes in humans [135]. The toxicity may be related to melanin binding. Drugs that bind to melanosomes can alter ion composition, especially calcium. Melanin may also have biochemical differences among species. Melanin in the RPE of hamsters is ultrastructurally different than the melanin in mice and this difference may account for interspecies differences in retinal toxicity [136].
Vigabatrin (gamma-vinyl GABA), an enzyme-activated, irreversible inhibitor of GABA transferase, is associated with visual disturbance in human patients. Patients treated with vigabatrin had peripheral field visual defects, but the macula was spared [137]. The precise pathology was unclear [138]. In a preclinical 90-day rat study, there was mild disorganization of the ONL and displacement of photoreceptor nuclei. These changes were more prominent in the periphery of the retina and occurred as dose-related findings in albino Sprague-Dawley rats, but not in the retina of pigmented Lister-Hooded rats [50, 137]. It is thought that vigabatrin is detoxified by melanin [139, 140].
< div class='tao-gold-member'>
Only gold members can continue reading. Log In or Register a > to continue
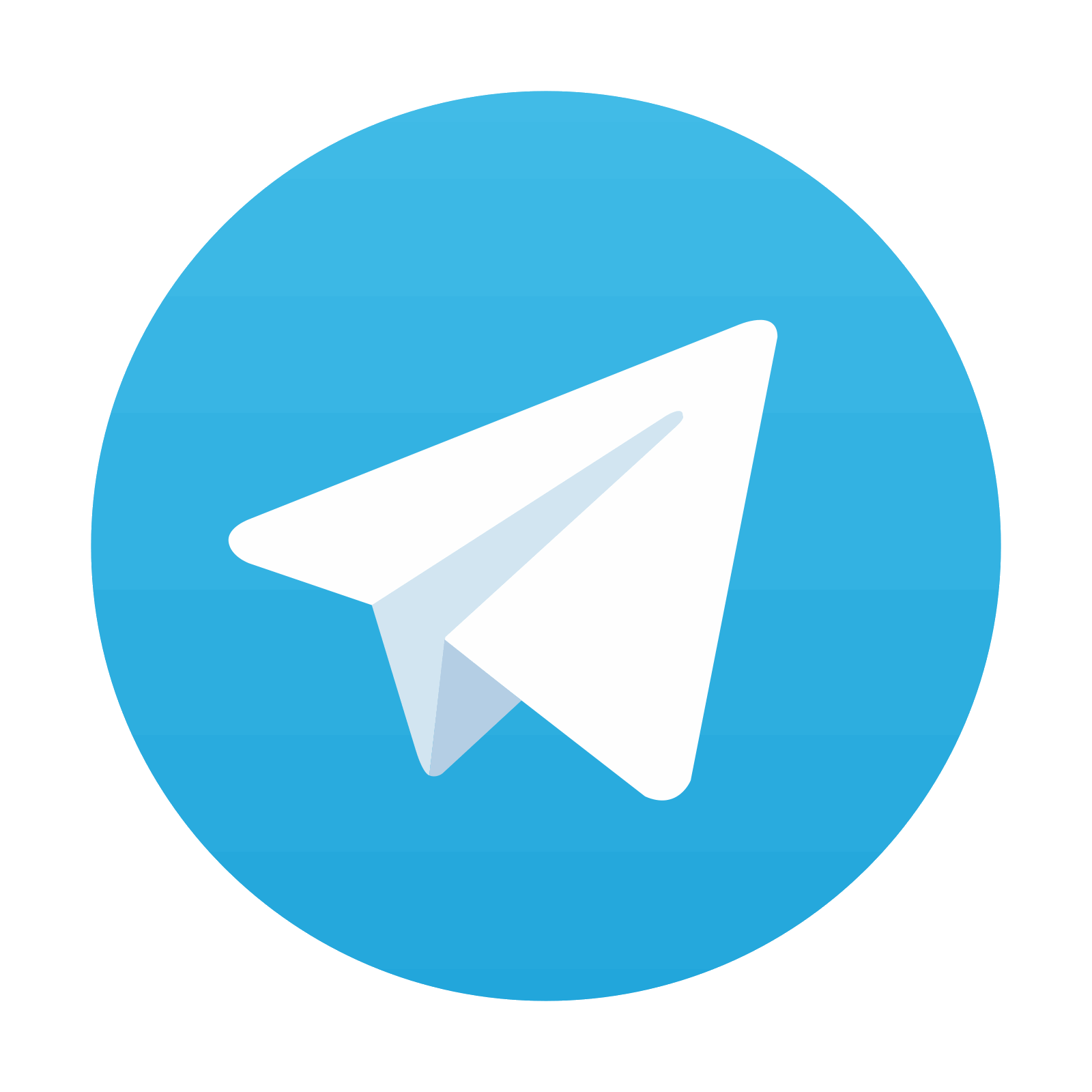
Stay updated, free articles. Join our Telegram channel
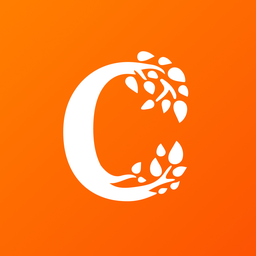
Full access? Get Clinical Tree
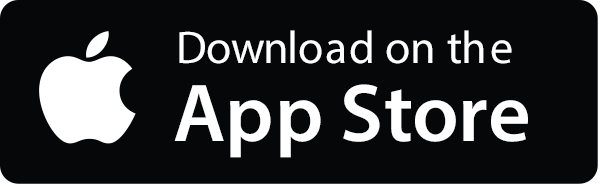
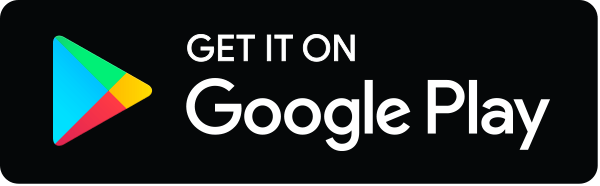