In canine performance, many variables, such as conformation, genetics, and behavioral drive, are major determinants for success, yet environmental variables such as training and nutrition are important as well. Athletic canine venues and events have increased in number in the past 20 years, with limited physiologic understanding of the requirements for the numerous activities in which dogs partake. There are considerable nutritional physiology data on the two athletic extremes: endurance dogs (sled dogs) and sprinting dogs (Greyhounds). Nutritional studies for more popular activities, such as agility and field trials, have been largely ignored, leading to attempts to translate endurance and sprinting knowledge into sound nutritional principles for all sporting dogs. These principles are addressed here, focusing on three areas: energetics, nutrition for performance, and nutrition during rehabilitation.
Energetics and the Myofiber
Conversion of Substrate to Energy
The generation of ATP is a universal process in all cells that occurs through the enzymatic machinery of the mitochondrial electron transport chain (ETC). In short, the ETC utilizes free hydrogen to create an electrochemical gradient between the inner and outer mitochondrial membrane that generates a covalent bond between ADP and inorganic phosphate to make ATP as the storage form of energy.
ATP generation and hydrolysis becomes a central theme to create appropriate muscle contraction. ATP is ultimately formed from the generation of hydrogenated nicotinamide adenine dinucleotide (NADH) and dihydrogenated flavin adenine dinucleotide (FADH2) in the citric acid cycle within mitochondria. The citric acid cycle is a complex of many enzymes that convert the by-products of glucose, protein, and fat degradation as pyruvate (glucose and some amino acids) and acetate (fatty acids and some amino acids) into covalently bound CoA moieties to make acetyl CoA, the major entry point substrate for the citric acid cycle (Figure 4.1). No matter where substrates enter the citric acid cycle, they are eventually turned into carbon dioxide, with oxygen as the reactant in the process for generating ATP (Shulman & Petersen, 2009).
Figure 4.1 The citric acid cycle showing acetyl CoA entry and the formation of NADH and FADH2 with the liberation of CO2 and GTP. NADH and FADH2 then enter the electron transport chain.

Glycolysis and Beta Oxidation of Fatty Acids
ATP generation occurs through beta-oxidation of fat and glucose oxidation through glycolysis, both of which lead to acetyl CoA production. Beta-oxidation of fat is a critical process to normal daily energy metabolism, as fat is considered the preferred fuel during rest. Glycolysis is a complex process that begins with fructose conversion to glucose or glucose immediate entry into the glycolytic pathway. This breakdown of glucose results in one 6-carbon molecule, generating two 3-carbon precursors, which eventually generate 2 NADH and 4 ATP and 2 pyruvate molecules (Figure 4.2). ATP is produced rapidly and does not require oxygen, which is the rate-limiting step in the citric acid cycle, making glycolysis a preferred system to generate ATP quickly and anaerobically. The excess pyruvate is converted to lactate under anaerobic metabolism. Glycogen stores for ATP generation, unlike fat in most cases, are limited.
Figure 4.2 Basic anaerobic glycolysis showing glucose being split into two pyruvate molecules which eventually become acetyl CoA for entry into the citric acid cycle. Note that two ATP are utilized, but four ATP are made in the reaction to provide quick anaerobic energy.

Glycolysis occurs in the cytoplasm of all cells. During rest, glycolysis is inhibited, causing a flux of glucose toward eventual glycogen formation (Figure 4.3). In times of need, increased glycogen phosphorylase activity induces glycogenolysis and generation of glucose 6-phosphate for glycolysis and entry into the citric acid cycle. Beta-oxidation of fat occurs in the mitochondria, and transport of free fatty acid into the inner mitochondria is required. Carnitine is bound to the carboxyl terminus of the free fatty acid in exchange for coenzyme A (Figure 4.4). Once the carnitine is bound, the fatty acid is transported across the outer mitochondrial membrane by carnitine acyl transferase I to the inner compartment, and then carnitine palmitoyl transferase II transports the fatty acid carnitine moiety into the mitochondria. The fatty acid becomes conjugated to acetyl CoA again, and then carnitine undergoes reverse transportation back to the cytoplasm (Shulman & Petersen, 2009).
Figure 4.3 Glycogenolysis occurs when excessive glucose is taken into cells as reserve glucose stores, and is eventually broken down for glucose when needed.

Figure 4.4 Fatty acid entry into the mitochondria is dependent on carnitine-mediated transport through both the outer and inner mitochondrial membranes via carnitine palmitoyltransferase 1 and carnitine acylcarnitine translocase. After fatty acid transport, carnitine is recycled through actions of carnitine palmitoyltransferase 2. Fat within the mitochondria will then undergo beta-oxidation and entry of acetyl CoA into the citric acid cycle.
Reproduced by permission of Hand et al. (2010).

Energetics of Substrates
The unit of energy generated by metabolism of protein, carbohydrate, and fat, is the calorie or joule. The kilocalorie (kcal) is the unit referred to in lay terminology when talking about calories, and is equivalent to 4.16 kJ. One gram of protein placed in a bomb calorimeter generates approximately 5.24 kcal. Fat generates 9.16 kcal/g and carbohydrate 4.26 kcal/g. This is the gross energy (GE) of the substrate and does not take into account the energy lost in feces due to incomplete digestion. This loss is reflected in the digestible energy (DE). Metabolizable energy (ME) as reported on pet food labels takes into account energy lost in urine, digestion, and gases. ME is assumed to be significantly less than the GE or DE. Protein and carbohydrate are generally given a value of 4 kcal/g, and fat 9 kcal/g as calculated by the Atwater equation when evaluating human foods (Table 4.1). This equation is modified to adjust for slightly less digestible ingredients in a manufactured pet food, making protein and carbohydrate approximately 3.5 kcal/g, and fat 8.5 kcal/g (National Research Council, 2006a, 2006b).
Table 4.1 Atwater’s formula and modified Atwater’s equations
Atwater’s formula |
Protein = 4 kcal/g × 24 = 84 kcal |
Fat = 9 kcal/g × 12 = 108 kcal |
Carbohydrate = 4 kcal/g × 50 = 200 kcal |
Total kcal = 404 kcal |
Modified Atwater’s formula |
Protein = 3.5 kcal/g × 24 = 84 kcal |
Fat = 8.5 kcal/g × 12 = 102 kcal |
Carbohydrate = 3.5 kcal/g × 50 = 175 kcal |
Total kcal = 361 kcal |
The above formulas demonstrate use of Atwater’s Formula and Modified Atwater’s Formula to calculate kilocalories in a pet food that consists of 24% protein, 12% fat, and 50% carbohydrates on a dry matter basis in 100 g. Note the modest differences between the two equations. The modified Atwater’s equation is used to calculate most commercial pet foods and can potentially undercalculate the number of kilocalories in highly digestible foods.
Body Condition and Energy Utilization at Rest and During Exercise
The National Research Council (NRC) has set forth energy and nutrient requirements for dogs. Two equations are used to represent resting energy requirement (Table 4.2). The linear equation closely mimics the results obtained from the exponential equation until the patient weight reaches 25 kg, when the linear equation diverges, overestimating the energy requirements needed at rest. A multiplication factor of 1.0–2.0 is applied to the linear equation’s initial calculation of (30 × kilogram body weight) + 70 which is considered resting energy requirement (RER) based on activity. The exponential equation is (kilogram body weight)0.75 multiplied by 70, which is considered the RER. For activity, this multiplication factor is increased up to approximately 140 to calculate maintenance energy requirements (MER) for active pet dogs. These equations are starting points for patients undergoing rehabilitation. When integrating exercise into a therapeutic plan, 20–30 minutes of rehabilitation activity three to four times a week would likely result in a negligible energy increase. This should be recognized when making a diet plan for a dog undergoing rehabilitation.
Table 4.2 Calculations for resting and active energy requirements
Linear equation for a 30-kg dog |
Resting energy requirement = 30 × BW (kg) + 70 = 970 kcal |
Active maintenance energy requirement = 2{30 (kg) + 70} = 1940 kcal |
Exponential equation for a 30-kg dog |
Resting energy requirement = 70 × BW × BW (kg)0.75 = 897 kcal |
Active maintenance requirement = 140 × BW (kg)0.75 = 1794 kcal |
Increasing physical activity and the effects of training have been extensively studied in dogs during treadmill exercise. The maximum oxygen consumption during exercise (VO2 max) reflects the energy that can be generated via oxygen utilization in the mitochondrial ETC. An average 20-kg Foxhound or Alaskan sled dog working at maximal oxygen consumption would need approximately 700–900 kcal/h of work based on the experimental conditions set forth in simulated treadmill exercise (Ordway et al., 1984; Musch et al., 1985; Reynolds et al., 1999). This oxygen consumption is directly related to skeletal muscle mitochondrial density and volume. In the field, there are many other factors to take into consideration, such as external temperature, thermal regulation, variability in terrain, and incline versus decline. Treadmill exercise results in a loss of efficiency in energy utilization with increased incline; the need for vertical rise increases overall kcal expenditure. Larger dogs need to exert more energy to break the fall that occurs on decline (Taylor et al., 1972; Ordway et al., 1984; Schmidt-Nielsen, 1984; Reynolds et al., 1999). Uneven footing or poor footing (snow and sand), as well as load bearing result in increased energy expenditure (Taylor, 1957).
Field studies examining energy expenditure of working Greyhounds have shown that the average 32–35 kg Greyhound expends approximately 2050–2160 kcal/day. These studies take into account the typical training regimen for a racing Greyhound, which includes being penned or caged with daily sprint training in enclosed paddocks for approximately 30 minutes and two races a week (Hill et al., 1999, 2000). These findings translate to approximately 150–160 kcal/kg0.75. This relatively high MER may be a reflection of the increased muscle mass in Greyhounds.
Interestingly, studies by Hill and colleagues suggested that feed restriction during racing from the normal of approximately 155 kcal/kg0.75 body weight down to a restricted regimen of only 137 kcal/kg0.75 resulted in decreased racing times, making mild feed restriction advantageous (Hill et al., 1999). There are numerous reports of caloric intake in racing sled dogs. Decombaz et al. suggested an intake of only 2100 kcal/day when dogs were running an average of 79 km/day (228 kcal/kg0.75), while Orr suggested an intake of approximately 4400 kcal when dogs were pulling a heavy load over ice 32 km/day (270 kcal/kg0.75) (Orr, 1966; Decombaz et al., 1995). These discrepancies appear significant and may be related to climate, housing, and activity in and outside of the kennel. Additionally, these estimates did not factor in changes in lean and fat mass during these activities.
Two studies used water double-labeled with deuterium and O18 (heavy oxygen) to assess total oxygen loss via urine versus that generated and lost through respiration as CO2. This technique allows calculation of relative CO2 loss, which is a rough estimate of total kilocalories consumed, since the only route for CO2 generation is the ETC. Dogs running an average of 79 km/day over 8 days expended approximately 438 kcal/kg0.75; however, the conditions and temperature during the field experiment were not reported (Decombaz et al., 1995). A second study by Hinchliff and colleagues in extreme racing conditions with dogs running at a speed of approximately 7 km an hour approximately 14 h/day over 5 days (total 490 km) at temperatures between −10 and −35°C suggested the average kcal expenditure in 18 dogs averaged 1052 ± 192 kcal/kg0.75 /day (Hinchcliff et al., 1997b). Metabolically, these dogs would need approximately 10,000 kcal/day to maintain body weight, suggesting many dogs in these conditions may utilize body reserves to meet the caloric demands since maintaining adequate intake is difficult.
Most performance dog owners are acutely aware of their animals’ body condition scores (BCSs) and competitive body weights. The traditional body condition scoring methods use a 5- or 9-point system. The 9-point system has been validated through comparison to dual X-ray absorptiometry analysis (Laflamme, 1997) and is preferred by the author (Purina, 2012). Typically, performance dog owners keep their dogs at a 4–5/9 BCS where ribs are easily palpable, there is an obvious abdomen tuck, and when viewed and felt from the top, a waist can be seen and the spinous processes can be felt. In athletic dogs, particularly Greyhounds, field trial, hunting, and sprint sled dogs, it may be more desirable to have a BCS between 3 and 4. In this paradigm, ribs can be visualized in shorter haired dogs, the spinous processes and wings of the ilea are prominent, but ample paralumbar musculature extends between the wings of the ilea so that the sacral spinous processes can be localized, but do not protrude. Dogs in sprinting and intermediate activity (10–30 minutes) need to be lean to achieve ideal performance (Figure 4.5), and restricted meal feeding is common. In endurance activities where speed is not as important, and there is a greater chance for body condition loss during extended activity, a BCS of 4–5 may be ideal before competition due to the potential for weight loss during competitive racing (Hinchcliff et al., 1998).
Figure 4.5 Appropriate body condition for a working sled dog, current BCS 4 of 9. Note that the musculature is defined and ribs are visible to the eye, which may not be the case in heavier coated dogs.

Nutrition for Performance
Dietary Protein: Beyond Energy
Although they must ingest essential amino acids (Table 4.3), dogs can synthesize nonessential amino acids through amidation, deamination, and carboxylation reactions that convert carbon precursors and essential amino acids into nonessential amino acids. Nitrogen retention studies rely on nitrogen balance as the measure of adequacy; however, most canine nitrogen retention studies have never examined the consequences on lean mass. The NRC states that only 20 g/1000 kcal of protein is required for maintenance, equating to approximately 10% crude protein in a typical dry commercial product.
Table 4.3 Essential and nonessential amino acids
Dietary essential amino acids | Dietary nonessential amino acids |
Isoleucine | Alanine |
Leucine | Tyrosine |
Lysine | Aspartic acid |
Methionine | Asparagine |
Phenylalanine | Glutamine |
Tryptophan | Glutamic acid |
Valine | Glycine |
Histidine | Serine |
Threonine | Cysteine |
Arginine | Proline |
Dietary protein helps maintain muscle integrity and appropriate total protein, albumin, and red blood cell status. The hematocrit and serum albumin tend to decrease with training and racing; this appears to be a result of overtraining syndrome (Kronfeld et al., 1989; Wakshlag et al., 2010). This syndrome can be correlated to the acute phase response whereby the liver changes its protein production profile to proteins such as haptoglobin and c-reactive protein, possibly at the expense of albumin (Wakshlag et al., 2010; Kenyon et al., 2011). The mechanism for these changes is poorly understood, but is thought to be due to acute inflammation caused by exercise with potential cytokine alterations resulting in decreases in red blood cell counts (Kronfeld et al., 1989; Wakshlag et al., 2010; Kenyon et al., 2011). Adequate protein intake may be helpful in ameliorating this condition.
Studies examining protein consumption and its role in maintaining red blood cell counts and hematocrit in training sled dogs have postulated that approximately 30% of the ME (70–80 g protein/1000 kcal) should come from highly digestible animal-based protein (Kronfeld et al., 1989). Four groups of sprint racing sled dogs exercising approximately 60 km in fieldwork per week, plus treadmill training each week, on four diets comprising 18% ME protein (48 g/1000 kcal), 24% ME protein (60 g/1000 kcal), 30% ME protein (75 g/1000 kcal), and 36% ME protein (90 g/1000 kcal) from an initial diet of approximately 26% ME protein were examined. After 12 weeks of feeding each diet, routine complete blood counts, serum chemistries, VO2 max, and physical assessment were performed. Six of eight dogs in the lowest protein diet (18% ME) sustained musculoskeletal injuries and showed a 25% drop in VO2 max. Dogs in the highest protein groups displayed a 10% increase in plasma volume, and there was a linear correlation between hematocrit, hemoglobin, and total blood volume and protein intake (Reynolds et al., 1999). Querengaesser and colleagues examined diets of approximately 72 and 85 g/1000 kcal and showed that there was no difference in the hematocrit decline over a 6-month period of training, but the higher protein group had elevated postexercise hematocrit, further suggesting that protein may affect parameters associated with performance (Querengaesser et al., 1994). An interesting study of mixed breed dogs exercised 4 h/day at 12 km/h compared soy protein versus fish- and meat-meal-based protein at approximately 35% of the ME. After 3 weeks, there was decreased hematocrit and increased red blood cell fragility in the soybean-meal-fed dogs (Yamada et al., 1987). These data suggested that endurance dogs should receive a minimum of 70 g/1000 kcal (approximately 26% of ME) of highly digestible animal-source protein with no upper limit yet defined.
In sprinting dogs, the picture may be slightly different as Hill and colleagues performed studies suggesting that racing Greyhounds perform better on lower protein diets of 63 g/1000 kcal versus 106 g/1000 kcal (Hill et al., 2001b). These diets substituted carbohydrates for the protein in an isocaloric exchange of nutrients; therefore, the enhanced performance may have been due to the increased carbohydrate in the diet, not the lack of protein. Though it appears that approximately 60 g/1000 kcal may be adequate for racing Greyhounds, further decreases in protein have not been evaluated and are very contrary to current feeding practices. Most Greyhounds are provided 0.25–0.5 kg of meat mixed with dry commercial dog food, approaching 106 g/1000 kcal to meet their energy requirements (Hill, 1998; Kohnke, 1998). The differences noted in sprinting dogs and endurance dogs may be related to overall muscle turnover rates, as exercise leads to increased protein turnover, and increased muscle inflammation is observed with increased duration of exercise (Wasserman et al., 1988, 1989, 1991; Wakshlag et al., 2002a). Without firm recommendations or studies in sprinting dogs, a reasonable recommendation is that most sprinting and intermediate activity dogs receive minimally 60 g/1000 kcal consumed (22–24% ME).
Dietary Fat and Carbohydrate: Energy and Demands
The respiratory quotient (RQ) is the ratio of oxygen consumed to CO2 generated. If the RQ is close to 0.7, primarily fat is being consumed for CO2 production, while if the RQ is closer to 1, primarily glucose is being consumed (Figure 4.6). Early in exercise (the first 20–30 minutes), protein oxidation is minimal; therefore, substrate utilization and changes in oxygen consumption can be examined when feeding differing carbohydrate and fat diets (Toll & Gillette, 2010). A significant amount of work has been performed examining gradation of exercise and duration as they relate to VO2 max and RQ values (Wagner et al., 1977; Granjean, 1998). These studies have laid the groundwork for our understanding of oxygen consumption, showing that when dogs utilize up to 40% of their VO2 max, they primarily utilize fat for energy, while between 40% and 70%, they utilize a mixture of glucose and fatty acids. Once an animal reaches 70% or higher oxygen consumption, they utilize primarily glucose for energy (Toll et al., 1992; Reynolds et al., 1995) (Figure 4.6).
Figure 4.6 Depiction of running speed versus the maximal oxygen consumption. Note that the VO2 max is reached well before maximal speed is reached reflecting anaerobic glycolysis generation of energy which can only be sustained for short periods of time. VO2 max is often reached at between 65% and 75% of maximal running capacity.
Reproduced by permission of Hand et al. (2010).

Animals working at maximal speed during the first few seconds of exercise rely upon immediate energy from the phosphocreatine system that generates ATP through shuttling inorganic phosphate to ADP. Glycogenolysis soon ensues, generating energy for only a few minutes. Generation of pyruvate and eventually lactic acid predominates if glycolysis is maintained, leading to pH alteration and intracellular dysfunction. Pyruvate incorporation into the citric acid cycle (carbohydrate oxidation) becomes a major source of energy for long-term exercise (from 20 minutes to 2 hours) as long as glycogen is present for glycogenolysis. Eventually, protein oxidation will take place if glycogen is depleted in endurance exercise. As glycogen is depleted, a dog will not be able to sustain oxygen consumption above 50–60% of VO2 max. Fatty acid oxidation begins to rise by 30 minutes of continuous exercise and will be sustained at an oxygen consumption rate of between 30% and 50% of the maximum as the primary fuel. This provides acetyl CoA production for the citric acid cycle at a steady rate, allowing some dogs to exercise at this low to moderate oxygen consumption rate for multiple hours (Reinhart, 1998; Toll & Gillette, 2010) (Figure 4.7).
Figure 4.7 The graph depicts the VO2 max based on duration of exercise. Note that initially, VO2 max is above 100% reflecting anaerobic energy generated by creatine phosphate reserves and glycolysis which eventually plateaus in 2–4 minutes with carbohydrate oxidation. As glycogen stores are depleted, beta oxidation of fat occurs and sustains the exercise period somewhere around 60–90 minutes, making fat the primary fuel for exercise in the endurance athlete.
Reproduced by permission of Hand et al. (2010).

This understanding provides the basis for fat use as a major form of energy in canid diets, which is contrary to recommendations in human endurance exercise, which focuses on carbohydrate loading (Hargreaves et al., 1984). It has been shown that time to exhaustion during low-intensity exercise did not correlate with glycogen depletion in dogs (Downey et al., 1980). The generation of energy from fat is up to 70% of the ME during long-duration exercise, suggesting a propensity for fat utilization that may be due to the dog’s high aerobic activity in skeletal muscle and increased mitochondrial density as compared to humans (Wakshlag et al., 2004). Beagles running at low to moderate intensity increased their time to exhaustion by approximately 25% when provided diets with 55–81 g/1000 kcal of fat versus 33 g/1000 kcal (Downey et al., 1980). Kronfeld, Hammel, and colleagues showed that dogs performed equally well on diets containing absolutely no carbohydrate compared to two diets with increasing carbohydrate content (Hammel et al., 1977; Kronfeld et al., 1977). Further studies in trained and untrained sled dogs showed that when comparing a high-carbohydrate (162 g/1000 kcal; 59% ME) low-fat (18 g/1000 kcal; 14% ME) diet to a high-fat (70 g 1000 kcal; 58% ME) low-carbohydrate (43 g/1000 kcal) diet, there was no difference in muscle glycogen storage (Wagner et al., 1977). Interestingly, the dogs on the high-fat diet showed diminished glycogen consumption with exercise. In endurance, huskies racing approximately 100 km/day over 5 days showed immediate glycogen depletion, with an increase in skeletal muscle glycogen and gradual depletion (Reynolds et al., 1995) of skeletal muscle triglyceride further suggesting that the longer these endurance dogs run, the more they adapt to fat utilization, sparing muscle glycogen (McKenzie et al., 2008).
Fat consumption can supply approximately 60–70% of the ME, and in times of extreme demand, fat may supply up to 85% of the ME, particularly in endurance sled dogs. Based on the previous studies, it is advised to introduce fats to the diet slowly. Adaptation to high-fat diets will take approximately 8–12 weeks to allow for mitochondrial and metabolic adaptation (Reynolds et al., 1994; Reinhart, 1998). This will help to prevent steatorrhea, which is a common occurrence when feeding high-fat diets. Excess fat in the diet may also require an increase in divalent cation nutrients (calcium, iron, zinc, copper, and manganese) due to soap formation with free fatty acids chelating these cations, making them less available for absorption, particularly calcium.
The amount of fat in the Greyhound diet is highly debated as studies by Toll and colleagues have shown that Greyhounds on a high carbohydrate diet (46% ME vs. 6% ME) were 0.4 km/h faster (Toll et al., 1992). The high-carbohydrate diets contained only 31% ME fat, while the high-fat diet consisted of 75% ME as fat. Hill and colleagues showed slightly different results, suggesting that Greyhounds fed 25% ME protein, 32% ME fat, and 43% ME carbohydrate performed better than those on a higher carbohydrate diet (21% ME protein, 25% ME fat, and 54% ME carbohydrate) (Hill et al., 1999). These results taken together with previous reports suggesting that higher carbohydrate diets enhance performance imply that approximately 30% ME fat and 24% ME protein with the remaining ME from carbohydrate seems adequate for racing Greyhounds. Surprisingly, this type of dietary breakdown results in a product that would be approximately 24–28% dry matter protein, 12–14% dry matter fat, and 45–50% carbohydrate, which is similar to many commercial adult pet foods on the market.
Very little information regarding optimal dietary fats for canine athletes is available, but there has been some speculation that chain length and saturation can affect a variety of issues from inflammation to oxidative potential during exercise (Bauer, 2006). Medium-chain triglycerides when digested liberate 8–12 carbon fatty acids that undergo some direct absorption into the bloodstream and are transported via albumin to cells for metabolism (Table 4.4). This has led to speculation that medium-chain triglycerides in the form of coconut and palm oils can be utilized more rapidly at the initiation of exercise leading to further glycogen sparing (Hawley, 2002; Jeukendrup & Aldred, 2004). This does not appear to be the case in other species, and one pilot study in athletic dogs showed limited utility; thus, it cannot currently be recommended as a strategy in fat adaptation (Reynolds et al., 1998). Discussion of polyunsaturated fatty acids will be reserved for rehabilitation where their influence on inflammation may be more pertinent. Since many performance animals including foxhounds, hunting dogs, and service dogs rely on detection capabilities, the potential use of polyunsaturated fatty acids in olfaction is of interest. A small study using only four dogs in a Latin square design showed that olfactory performance appeared to be enhanced when dogs were provided a base diet in which dietary fat sources were switched from animal-based fat to corn oil-based fat. Corn oil has extremely high concentrations of the polyunsaturated fatty acid linoleic acid, which may enhance olfactory capability in scent-trained Labradors (Altom et al., 2003). Another study examining hunting dogs suggested that a diet with slightly higher protein and higher fat improved bird find rates (Davenport et al., 2001). Whether this was due to the altered substrates, differences in ingredients, or long-chain polyunsaturated fat enrichment could not be determined based on the experimental design.
Table 4.4 Differences between medium- and long-chain triglycerides
Medium-chain triglycerides | Long-chain triglycerides | |
Chain length | 10–14 carbons long | 16–22 carbons long |
Kilocalories per gram | Approximately 7 kcal | Approximately 9 kcal |
Primary GI absorption | Direct enterocyte to portal blood | Synthesis into chylomicrons |
Transport in blood | Carried via albumin | Chylomicrons and lipoproteins |
Cell absorption | Diffusion | Lipase dependent absorption |
Carbohydrates: Timing and Strategy
The use of carbohydrates as a major dietary substrate makes sense in sprinting animals like Greyhounds with approximately 40–50% of the ME in the diet as highly digestible carbohydrates. Endurance sled dogs need less than 10% of the ME as carbohydrates, as there are no definitive carbohydrate requirements in these dogs (Reinhart, 1998; Hill et al., 2001b).
Carbohydrate loading is a principle used in human athletics, which can translate into the canine arena. It may be beneficial for sprinting and intermediate athletes, particularly over multiple day events, where muscle glycogen is depleted daily and needs to be replenished (Reynolds et al., 1997; Wakshlag et al., 2002b; McKenzie et al., 2008). Studies performed in sled dogs have definitively shown that postexercise supplementation with a maltodextrin supplement at 1.5 gm/kg BW within 30 minutes of exercise increases skeletal muscle glycogen within 4–24 hours (Hinchcliff et al., 1997a, 1997c). In both studies, it was evident that this dosing returned muscle glycogen to baseline concentrations before exercise the following day, while without supplementation, skeletal muscle glycogen content was only 50% of baseline concentrations (Reynolds et al., 1997; Wakshlag et al., 2002b). Based on this information, postexercise carbohydrate repletion is recommended in dogs running anywhere between 5 minutes and 3 hours per day, particularly when expected to perform activities that will facilitate carbohydrate oxidation similarly the following day. The effectiveness of this strategy in endurance events where dogs are running at a slower pace for extended periods of time is unknown, so this strategy is not routinely recommended.
In the human athletic arena, protein with carbohydrate is often provided postexercise. The protein is thought to help curb skeletal muscle proteolysis after intermediate exercise (Betts & Williams, 2010). This approach has not been examined in the canine performance arena. In human athletics, the use of whey-based protein in young athletes postexercise appears to help in retention of lean mass and possibly improves glycogen repletion in some situations (Betts & Williams, 2010), but firm recommendations in performance dogs cannot be given at this time.
Dietary Fiber
Dietary fiber, regardless of its form, leads to fecal bulk. This increase in fecal bulk can lead to inappropriate defecation during competition and extra weight carried by the competitor, which may be negative. Fiber comes in two forms; insoluble (nonfermentable) and soluble (fermentable). Insoluble fiber results in fecal bulk and acts as a binding agent that can improve fecal quality when diarrhea is a problem. Soluble fiber has the capacity to alter the large intestinal microflora and potentially increase the absorptive surface of the small and large intestine through villous hypertrophy. This has been used strategically in canid athletes with stress-related diarrhea. Soluble fiber tends to be a matrix on which certain bacterial families, including Bifidobacteria, Lactobacillus, and Streptococcus, thrive (Wakshlag et al., 2011; Gagné et al., 2013). These bacterial families ferment soluble fiber sources and liberate volatile fatty acids (acetate, butyrate, propionate) that promote colonocyte regeneration and may improve recovery from diarrhea (Whelan & Schneider, 2011). Many of the enteric formulas of commercial dog food use small amounts of gums, soy fiber, fructooliogsaccharides, other oligosaccharides, and mixed insoluble and soluble fiber sources, to improve fecal quality and intestinal absorptive capabilities. The amount of soluble fiber added is generally less than 2% of dry matter in the diet since overfermentation can result in deteriorating fecal quality (Beloshapka et al., 2011). Mixed soluble and insoluble sources commonly added to commercial dog foods to improve fecal quality are chicory root, beet pulp, tomato pomace, and psyllium. In many instances, the addition of psyllium husk powder to feed is used in exercising canines to improve exercise-related stress diarrhea. Psyllium husk fiber is unique since it is a mucilage with water binding properties, and much like insoluble fiber, provides a modest fermentation substrate. It is often recommended to start with approximately 4 g of psyllium (1 rounded teaspoon of fine powder) per day, titrating upward, not exceeding 16 g/day in a typical 20–30 kg canine athlete (Leib, 2000).
Electrolytes, Minerals, and the Canine Athlete
Minerals can be classified into major minerals and trace minerals (Table 4.5). The major minerals are of most importance since deficiencies in dogs fed nontraditional diets (meat base without bone) have been observed. If meats are being used, it is often advised to have the bones ground into the meat supply to improve the calcium and phosphorus balance. Calcium should be between 0.6% and 1.2% dry matter, with similar amounts of phosphorus to maintain calcium homeostasis for structural integrity of bone and appropriate cellular signaling and buffering capacities (National Research Council, 2006c). Magnesium is also a concern when bone is not a constituent in a nontraditional diet, and commercial feed is not being used. Deficiency can manifest in exercising dogs as hyperextension of the carpus; this has been observed in endurance huskies. Deficiencies of the other major minerals including sodium, potassium, and chloride have never been observed in adult working dogs; however, nonclinical hyponatremia has been observed in endurance huskies during races and simulated endurance races (Hinchcliff et al., 1993, 1997a, 1997c; McKenzie et al., 2007). It is believed that due to the high calorie meat consumption and high water turnover rate during these strenuous exercises, sodium conservation is heightened by increases in the renin/aldosterone/angiotensin system, as well as modest sodium loss causing a mild hyponatremia (Hinchcliff et al., 1997a, 1997c). This chronic hyponatremia was observed in the Yukon quest in 1992 and simulated endurance events in dogs from a specific kennel (Hinchcliff et al., 1997a, 1997c; McKenzie et al., 2007). Studies examining this phenomenon in three teams participating in the Race to the Sky in 2006 suggest that each team responded differently with one team showing no signs of subclinical hyponatremia (Wakshlag et al., 2010). This suggests that these shifts in sodium retention may be directly related to dietary sodium availability rather than being a unique phenomenon in endurance sled dogs. These findings do not warrant use of electrolyte mixtures to sustain dogs that are eating commercial rations since the only studies using such mixtures have shown no beneficial effects and/or potential gastrointestinal upset (diarrhea) after a day of activity (Young et al., 1960; Mazin et al., 2001). Most importantly, if providing sufficient commercial dog food, supplementation with any of these major minerals is not necessary. Furthermore, it should be noted that calcium availability will be hindered when providing fat at over 60% of the ME in a feed. Competitions where aggressive fat supplementation is used are often short duration events and should not affect long-term calcium homeostasis.
Table 4.5 Essential major and trace minerals
Major minerals | Trace minerals |
Potassium | Iron |
Calcium | Zinc |
Phosphorus | Copper |
Sodium | Manganese |
Chloride | Iodine |
Magnesium | Selenium |
Trace mineral intake (Table 4.5) will increase proportionally with intake of commercial dog food and will also increase to a lesser degree when using meat to supplement commercial diets. To date, there has not been an observed clinical deficiency in copper, zinc, iron, manganese, iodine, or selenium in athletic canines fed traditional commercial dog foods or commercial dog food/meat mixes. This is likely due to the fact that the amounts of trace minerals incorporated into most pet foods are greater than the minimum amount legislated by the Association of American Feed Control Officials (AAFCO), and also that athletic dogs have increased intake of food. Currently, it is unknown whether supplemental trace minerals are needed, or whether the amounts deemed sufficient for normal dogs will meet the demands of performance canids.
Vitamins/antioxidants and the Canine Athlete
Vitamins are classified as either fat or water soluble (Table 4.6). Water-soluble vitamins are involved in metabolism as intermediates and coenzymes within the citric acid cycle or as carriers and coenzymes for carbon transfer. Sufficient quantities of these vitamins are absolutely required for metabolism. Many commercial dog foods and meats tend to be fairly rich in these vitamins. Most commercial pet food will contain 2–10 times the minimum requirement since the water-soluble vitamins have large margins of safety. Vitamin C is the most abundant water-soluble antioxidant found in the mammalian body, and dogs sustain their requirements through hepatic synthesis; however, dogs may not synthesize as much as other species (Chatterjee et al., 1975). Lack of high hepatic synthesis, in addition to the fact that serum ascorbic acid concentrations decreased more than 50% after an undefined race distance of 1.5-hour duration, has led experts to speculate that supplementation may be useful (Kronfeld et al., 1989). Similar decreases have been observed in unsupplemented exercising Greyhounds (1.8–2.8 mg/L) (Marshall et al., 2002; Scott et al., 2002). Supplementation of 1 g of ascorbic acid a day returns serum concentrations closer to what is considered a normal baseline concentration (5–6 mg/L), but similar supplementation in Greyhounds for 4 weeks resulted in slower racing times by 0.3 km/h. Therefore, vitamin C supplementation, having shown no definitive benefit for athletic canines, is not routinely recommended at this time, particularly for racing Greyhounds.
Table 4.6 Essential water-soluble and fat-soluble vitamins
Water-soluble vitamins | Fat-soluble vitamins |
Thiamin (B1) | Vitamin A |
Riboflavin (B2) | Vitamin D |
Niacin (B3) | Vitamin E |
Pantothenic acid (B5) | Vitamin K |
Pyridoxine (B6) | |
Folic acid | |
Cobalamin (B12) | |
Choline |
Fat-soluble vitamins (A, D, E, and K) have smaller margins of safety and are more concerning. Sufficient vitamin K is synthesized by indigenous bacteria in the gastrointestinal tract of normal dogs. Vitamin A may be of concern if organ meats are used as a portion of the diet; they should not exceed 15% of any meat mix used to feed because of the high concentration of vitamin A found in liver. Excess vitamin A consumption may manifest as poor coat quality, perturbed bone mineralization, and hepatic damage. In general, however, dogs are very tolerant to high dietary vitamin A (Morris et al., 2010). Vitamin D is also found in organ meats, particularly liver, making small amount of organ meat desirable if using meat as part of the diet. Many commercial dog foods have at least twice the cholecalciferol needed in the diet, so with the increased energy consumption required by athletic dogs, the amount of cholecalciferol consumed is usually adequate.
Vitamin E is sufficient in commercial pet food, and most manufacturers add significantly more than the requirements, making vitamin E deficiency unlikely. Deficiency has been observed in hunting dogs fed an all-meat diet and was associated with retinal degeneration (Davidson et al., 1998). Vitamin E has been examined extensively in endurance sled dogs, and low serum vitamin E was associated with an increased risk of being dropped from the Iditarod. Additionally, serum vitamin E dropped after a single day of endurance activity in two separate studies (Kronfeld et al., 1989; Piercy et al., 2001a, 2001b). Increased serum creatine kinase was never directly correlated to low vitamin E concentrations in dogs exhibiting exertional rhabdomyolysis (Piercy et al., 2001a). Similarly, decreased serum vitamin E concentrations have been observed in Greyhounds racing 500 m (Scott et al., 2001). These finding suggested that supplementation might be beneficial. A consensus statement made by the Iditarod trail committee in 1996 stated that endurance sled dogs should be supplemented with 400 IU of vitamin E daily during training and racing in an effort to reduce exertional rhabdomyolysis; however, there appears to be no decrease in the incidence of exertional rhabdomyolysis (Iditarod Veterinary Trail Committee, pers. comm.). More compelling evidence for not supplementing vitamin E was provided when Scott and colleagues and Hill and colleagues showed that supplementing 100–1000 IU raised serum tocopherol concentrations, but that dogs receiving 1000 IU had slowed racing times (Hill et al., 2001a; Scott et al., 2001). Tocopherol supplementation is not recommended in sporting dogs as long as they are being fed sufficient complete and balanced dog food, while vitamin E supplementation (200–400 IU for a typical athletic 20- to 40-kg dog) should be considered for dogs being fed nontraditional diets (primarily meats) to prevent deficiency.
Some hypothesize that antioxidant cocktails have the ability to quench free radical damage more effectively than single agents, and there have been two separate studies examining this concept. The first study used a mixture of 475 IU of alpha tocopherol, 706 mg of vitamin C, and 5.1 mg of beta carotene per kilogram of food for 3 weeks. The other study used 400 IU of alpha tocopherol, 3 mg of beta carotene, and 20 mg of lutein per kilogram of food for 4 weeks (Baskin et al., 2000; Piercy et al., 2000). Both studies found no association with decreased muscle damage as evaluated by creatine kinase and limited antioxidant potential during recovery, while serum concentrations increased for all of the supplemented antioxidants. Other single agent studies examining astaxanthin (a carotenoid) and blueberries (flavonoids) have shown increased antioxidant potential in serum with no association with improved performance (Dunlap et al., 2006; Reynolds et al., 2010). Based on the limited information and lack of benefits for performance, supplementation is not recommended at this time.
Feeding Strategies in Canine Athletes
Feeding patterns can affect performance. Frequency and time of feeding become important to not only decrease fecal bulk, but also maximize metabolites that are typically used for the activity. Sprinting dogs running for less than 10 minutes during a single bout of exercise will benefit from modest feed restriction 24 hours prior to exercise (decreasing total meal by 20%) during single day events to decrease fecal bulk. Although some advocate small carbohydrate meals before exercise to provide glucose as fuel for impending exercise, there are few data to support this strategy in dogs (Hawley et al., 1997). Sprinting and intermediate athletes, particularly agility and field trial dogs, for example, that perform multiple bouts of exercise in a day, may benefit from immediate postexercise carbohydrates in small amounts immediately after a bout of exercise when expected to undertake another bout within 2–3 hours. If repetitive bouts are close to together, this may not be advised to avoid vomiting or regurgitation (Hill, 1998). During multiple days of competition, postexercise glycogen repletion is advised within 30 minutes of the last bout of exercise for the day to replenish muscle glycogen.
Intermediate athletes exercising once a day for 20–120 minutes typically rely on both glycogen and fat for energy generation; therefore, it is recommended that they be fed diets moderate in fat (50% of ME) and carbohydrate (20% ME), and high in protein (30% ME). This diet allows adequate muscle glycogen repletion during training and helps increase mitochondrial volume. Fat will be used as a primary fuel at rest and within 20 minutes of exercise allowing for glycogen sparing when these athletes are asked to run above 60% of VO2 max. These athletes will benefit from postexercise carbohydrate supplementation to restore muscle glycogen concentrations particularly during multiple day events (Reynolds et al., 1997; Wakshlag et al., 2002b). In an effort to promote fat use and lipolysis, feeding a single meal each day may be advantageous with that meal being fed about 2 hours postexercise. Modest feed restriction (20% of meal) the day before racing will prevent defecation during exercise, promote lipolysis, and decrease fecal bulk (Hill et al., 1999; Toll & Gillette, 2010). Care should be taken to not feed larger meals immediately after exercise particularly in larger deep-chested breeds prone to gastric dilatation and volvulus.
Endurance athletes (i.e., foxhounds and sled dogs) tend to be fed one or two large meals each day during training, which will typically need to be approximately 300–500 kcal kg0.75 during heavy training (Ordway et al., 1984; Musch et al., 1985; Decombaz et al., 1995; Hinchcliff et al., 1997b). These meals should be approximately 30% ME protein, 60–70% ME fat, and negligible carbohydrate (less than 10% ME). The ration will likely comprise over 50% commercial dog food, with the rest as high-fat meat. This is necessary to achieve the caloric density and digestibility needed for competitive racing and hunting. As training intensifies, there will be back-to-back runs of 60 mi or greater on different terrains thus increasing caloric demands, which are often met with high-fat meats in small chunks during rest periods on the trail; little is known about the nutrient composition, but these tactics appear successful during competitive endurance sled dog training and racing. During racing, dogs will be fed significantly larger meals during 4- to 8-hour or longer rest stops. Since carbohydrate is not a primary fuel for exercise in these athletes, postexercise carbohydrate supplementation for glycogen repletion is not recommended. Foxhounds that course through fields every day and pointing dogs asked to hunt for multiple hours may benefit from postexercise carbohydrates since they will rest for significant times (greater than 8 hours) between exercise bouts.
Feeding primarily meat is not routinely recommended for dogs due to the incomplete nature of this food, yet it is commonly practiced and is likely not detrimental for short periods of time (1–2 weeks). Typically, meat is provided raw, but most veterinary professionals recommend cooking the meat. Cooking does not appear to decrease digestibility significantly (Kerr et al., 2012). The major biologic difference between raw and cooked meat is pathogen contamination that can cause detrimental health effects in owners and dogs when feeding raw meat (Chengappa et al., 1993; Morley et al., 2006; Kukanich, 2011). Studies examining therapy dogs in Ontario have revealed that zoonotic pathogen exposure risk is approximately sevenfold higher for salmonella and 14 times higher for enterogenic antibiotic-resistant Escherichia coli species (Lefebvre et al., 2008). This risk has prevented raw-fed therapy dogs from being certified by the Delta Society at the time of writing. In addition to the zoonotic risk, the effects of high salmonella burden have led to the demise of Greyhound puppies and increased the risk of diarrhea in adult Greyhounds when using poor quality raw meats (Chengappa et al., 1993). There have also been salmonella outbreaks associated with commercial foods (Schotte et al., 2007; Behravesh et al., 2010), but the relative risk and level of contamination is far greater in raw meats; therefore, cooking is advised (Freeman & Michel, 2001; Weese et al., 2005).
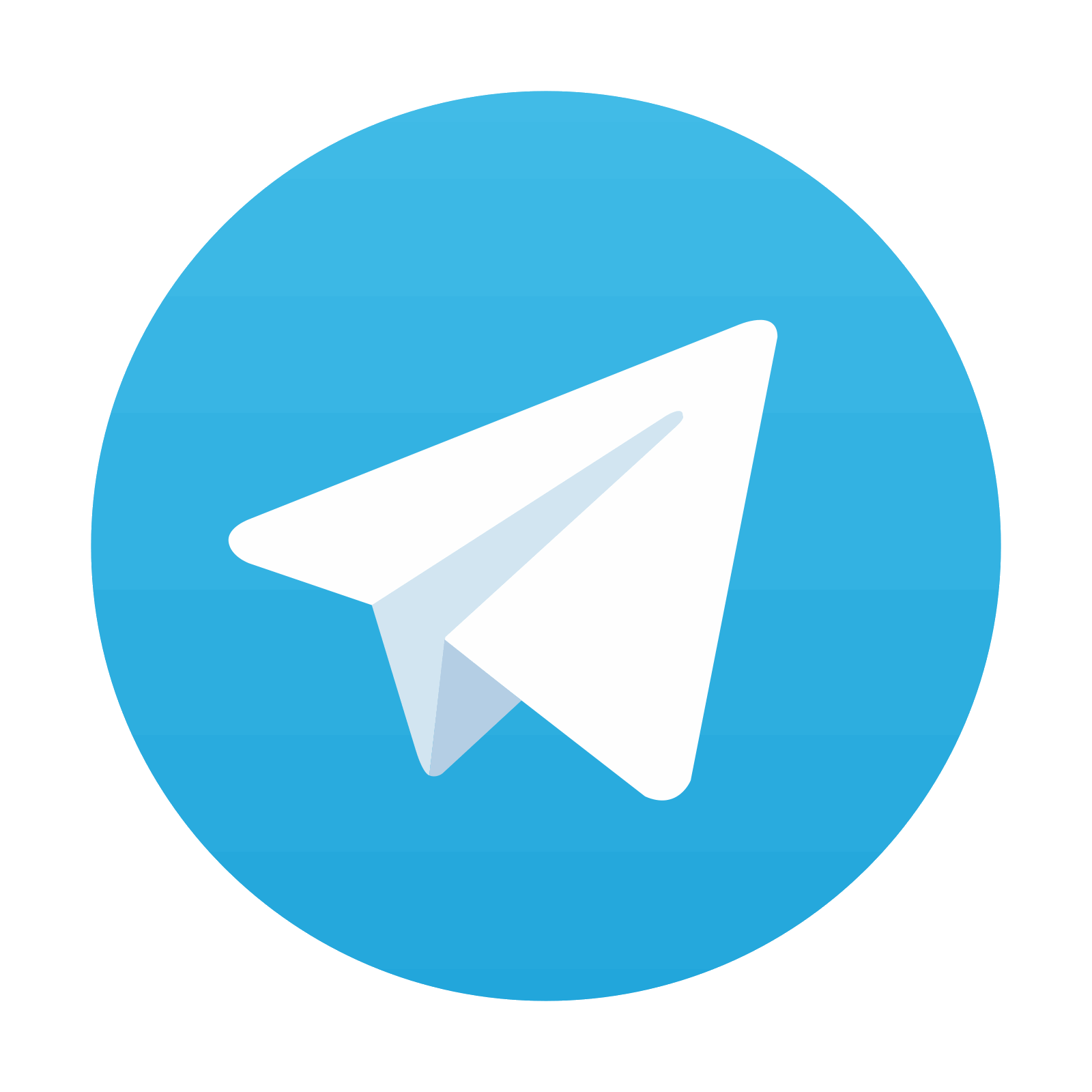
Stay updated, free articles. Join our Telegram channel
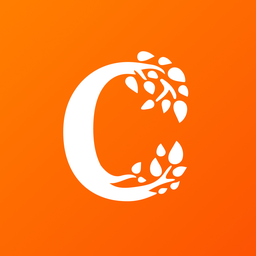
Full access? Get Clinical Tree
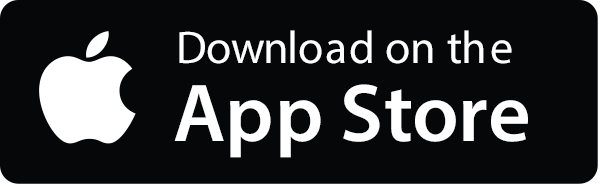
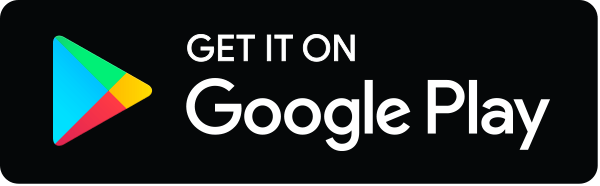