MELISSA BAIN AND C.A. TONY BUFFINGTON Animals interact with their environments on a time constant of milliseconds. When animals act on their environment, their environment responds (Fig. 4.1). Animals perceive this response through variable combinations of their senses, which transmit the received information chemically, electrically, and hormonally to various parts of the brain. The brain then compares the received information with predictions it makes based upon its history (genetic, epigenetic, and environmental), the context of the interaction, and its expectation (predicted response versus received response) and decides on the next action (Pezzulo et al., 2018). Fig. 4.1. A simplified representation of environmental stimuli processing by animals. External and internal stimuli activate variable combinations of sensors, which transduce the stimuli into signals interpretable by the central nervous system (CNS). The CNS creates prediction perceptions based on input, which are then compared with subsequent input to determine responses and their associated affects. Responses vary based on the nature of the stimuli and the animal’s reaction types. (Adapted and redrawn from Colditz and Hine [2016], used with permission.) Animals must be able to perceive environmental events as either threats or opportunities to survive long enough to transmit their DNA to the next generation. In a world containing more threats than opportunities, animals have developed a number of physiological and behavioral responses to environmental threats; which pattern of responses gets deployed seems to depend upon the ratio of the animals’ perception of control to its perception of threat (pC/pT) in its environment. When this ratio becomes less than one, the brain activates the central threat response system (CTRS), which directs variable combinations of its downstream effector systems to mount an appropriate response to the threat. Acute activation of the CTRS can restore the animal’s homeostasis, albeit sometimes requiring a change in activities of the threat response systems (‘allostasis’; McEwen and Wingfield, 2003) Chronic activation of the system, however, can negatively affect both health and welfare (‘allostatic overload’; McEwen, 2017a). We first define health to address the relationship between mental and physical health. In 1948, in the wake of WWII, the World Health Organization stated as the first principle of its constitution that, ‘Health is a state of complete physical, mental and social well-being and not merely the absence of disease or infirmity’ (World Health Organization, 1948). Although the definition was applied to humans, and has been critiqued by others (Witt et al., 2017), it seems to be a reasonable starting place for a definition of mental and physical heath for mammals in general. Broom (2006, p.75) observed that views on the concept of health differ among food animal producers, ethologists, and some veterinarians. He observed that, ‘…for most people, health refers to the state of the body and brain in relation to the effects of pathogens, parasites, tissue damage or physiological disorder’, that is, physical pathology. Studies of confined animals however – in zoos, production facilities, and people’s homes – have found that the quality of the environment also can affect animals’ mental and physical health. Defining the concept of ‘mental health’ is particularly tricky, since mental processes appear to be an emergent property of brains that cannot (yet) be measured directly, but rather might be inferred from the behavior of animals, or potentially from evaluation of pertinent biomarkers (see Section 4.4). Just as organ functions are emergent properties of their cell biology, we perceive mental processes to be generated from and dependent upon neural activity (Anderson, 1998), but nonetheless separate from it (Voneida, 1998). From this perspective, mental health implies brain health, so health results from variable contributions of genetic, epigenetic, and environmental interactions on animals. The challenge for writers of chapters that span these domains is daunting; to paraphrase Kozak and Cuthbert (2016, p.292), ‘Behavioral science studies what the brain does; genetics, cell and molecular biology, endocrinology neuroscience, immunology and physiology study how the brain does it’. Behaviors often associated with poor mental health include defensive aggression, withdrawal, hiding, and sickness behaviors, whereas those often associated with good mental health include play, exploration, and the like; the specifics of which may depend on the animal’s species and environmental context. Although behaviors may appear maladaptive when they develop secondary to a physical condition, one must be careful to avoid thinking of all behaviors associated with poor health or welfare as inappropriate, maladaptive, or malfunctional. For example, piloerection and lack of grooming are adaptive sickness behaviors that contribute to greater well-being by increasing insulating ability and water conservation, and decreasing heat loss and energy expenditure. And the increase in metabolic energy expenditure during a febrile episode can be made up later, after the inappetence due to lack of desire to hunt or forage for food that occurs during this episode has subsided, which may have served to protect the animal from predation (Hart, 2010, 2011). The word ‘stress’ means different things to different people (Del Giudice et al., 2018). In the context of mental and physical health, we define stress to mean effects of the (internal and external) environment on the organism. The eminent neuroendocrinologist and stress scientist Bruce McEwen (2017b) describes three categories of CTRS responses: good, tolerable, and toxic. Good CTRS responses, defined as brief and mild-to-moderate in magnitude, occur when an individual’s pC/pT permits it to perceive opportunities, take risks, rise to challenges, and feel rewarded by (often) positive outcomes. These responses are part of normal development and help develop threat coping skills when they occur in the safe, predictable environments with stable and supportive relationships. Examples of events resulting in positive responses in young companion animals include non-threatening visits to the veterinarian and exposure to novel situations and foods. These responses will be discussed further in the discussion on resilience (see Section 4.3). Tolerable CTRS responses occur with exposure to experiences that present greater threats, such as boredom or environmental instability (as may be seen, for example, by pet animals in a disruptive or chaotic household), serious illness or injury, or exposure to a natural disaster. As with positive responses, when these events occur in an otherwise safe environment, recovery to normal is likely. Thus, the essential characteristic that makes this level of threat intensity tolerable is the extent to which protective surroundings permit adaptive coping and a sense of control. In the most threatening environments, where the animal’s perception of threat exceeds its perception of control, a toxic CTRS response can result (McEwen, 2017a). Toxic CTRS responses, especially when frequent or sustained, are the most dangerous to the animal’s long-term health and welfare. Examples of early life threats that can result in toxic CTRS responses include severe nutritional deprivation (Bouret et al., 2015), separation (Lippmann et al., 2007), or life-threatening maternal or personal threats. When sustained, it is this level of perception of threat that is most damaging to mental and physical health (National Scientific Council on the Developing Child, 2005/2014). And what of well-being? The National Institute of Food and Agriculture states that ‘animal well-being is a complex topic’ (Anonymous, 2016). In addition to optimizing their list of, ‘…environmental conditions, such as temperature, humidity, duration of daylight, bedding, size and shape of living quarters, or the number of other animals sharing an environment’, we add the ability to engage in species-typical behaviors and to maintain a positive pC/pT. Understanding the relationships between mental and physical health also requires consideration across levels of analysis. For animals, these include the interactions of the macro and micro environments with the individual’s genetic, epigenetic, molecular, cellular, circuit, physiological, and behavioral domains. These relationships are depicted in Fig. 4.1. Genes obviously play a role in animals’ mental and physical health. The publication of many mammalian genomes during the past two decades has led to the hope that genetic causes for many chronic diseases would be identified, permitting more personalized disease treatment and prevention strategies. Unfortunately, most genome-wide-association studies in humans have failed to find large genetic effects (Rappaport, 2018). Genetic relationships between health and disease are complex and appear to result from relatively small effects contributed by many genes. Although we cannot yet directly treat gene-related health problems, they need to be understood. For example, diseases such as syringomyelia in Cavalier King Charles spaniels may present as a primary behavior problem of ‘compulsive circling’. Other genetic influences, such as the propensity for hip dysplasia, may directly affect animals’ quality of life. Painful conditions, and potentially the effects that exercise restriction exert, also can negatively affect an animal’s welfare. Moreover, some breeds are predisposed to display specific abnormal behaviors, such as the propensity for doberman pinschers to display the repetitive behavior of self-sucking. There is evidence for a genetic linkage to these behaviors, whether due primarily to behavioral predispositions or to a malformation of the brain (Dodman et al., 2010; Ogata et al., 2013). In addition to genetic influences on the physical health of animals, genes also can directly affect behavior. For example, animals bred to particular conformational standards, such as corkscrew tails in English bulldogs or polledness in Hereford cattle, may have difficulties communicating with a conspecific. If their signaling is misinterpreted by other animals, it could result in displays of aggression, some of which could directly affect their welfare, or the welfare of others in their groups. In addition to the individuals’ genetics (G), their mental and physical health is influenced by the environmental (E) influences they are exposed to during life (the ‘exposome’; Wild, 2005) plus their gene–environment (G–E) interactions. Rappaport (2016) recently used published data from Western European monozygotic twins to estimate the proportion of disease risk attributable to G (plus shared E) for 28 chronic diseases by calculating ‘population attributable fractions’ (PAFs). He reported that genetic PAFs ranged from 3.4% for leukemia to 48.6% for asthma, with a median value of 18.5% and interquartile range of 9.9% to 24.2%. Cancers had the lowest PAFs (median = 8.3%), whereas neurological (median = 26.1%) and lung (median = 33.6%) diseases had the highest PAFs. The effects of G and shared E exposures on development of the chronic diseases studied tended to be modest, with 75% of the phenotypes having PAFs less than 25%. Only two G-related PAFs were greater than 40%: thyroid autoimmunity (42%) and asthma (49%). McEwen (2017a) has also recently reviewed evidence showing that different alleles of commonly occurring genes within the CTRS influence how individuals will respond to environmental experiences. Moreover, while these alleles may exacerbate the negative effects of threatening environments on mental health outcomes and thus be labeled ‘bad genes’, ‘reactive’ or ‘context-sensitive’ may be better descriptors because in positive, nurturing environments these same alleles may lead to successful outcomes, particularly during development (Boyce, 2016). A momentous recent advance in our understanding of the origins of mental and physical health occurred with the documentation of the developmental origins of health and disease (DOHaD; Hanson and Gluckman, 2015). Although the effects of early life events on health and disease had long been surmised, recent developments can be traced to David Barker’s 1990 hypothesis of the fetal and infant origins of adult disease (Barker, 1990). Since then, examples of the effects of early life events on health, disease, and productivity have been reported in cats (Buffington, 2009), livestock (Reynolds et al., 2010; Gotoh, 2015) rodents (Anacker et al., 2014), and humans beings (Anda et al., 2006). Evidence from clinical, epidemiological, and experimental observations has shown how evolutionarily conserved developmental processes can interact with environmental cues, often transmitted from the mother via the placenta to the offspring, to attempt to match the physiology of the fetus to its postnatal environment. The sequence of events that has emerged from this research proposes that when a pregnant female is exposed to a sufficiently harsh and salient threat, the neuroendocrine products resulting from activation of her CTRS can cross the placenta and affect the course of fetal development (Meaney et al., 2007; Cottrell et al., 2014). The biological purpose of transmitting environmental cues to the fetus may be to guide the development of the fetal CTRS and associated behaviors to increase the probability of survival (Matthews, 2002; Gluckman and Hanson, 2006a). As Gluckman and Hanson (2005) described, the fetus may use cues from its intra-uterine environment to make predictive adaptive response ‘decisions’. In a practical sense, if a threatening or nutrient-limiting environment is perceived, the developmental trajectory of the fetus may change in response to the available information to enhance reproductive fitness in the predicted environment. Sensitizing the CTRS may be part of a more general ‘survival phenotype’ that includes smaller (or larger) size at birth (Parlee and MacDougald, 2014). Although the phenotype does not appear to affect reproductive capacity, it has been associated with a variety of adverse health outcomes. Studies of the enduring effects of stressful developmental experiences on health have now been published in a wide variety of mammalian species, including rodents (Molet et al., 2014), carnivores (Buffington, 2011), food production animals (Reynolds et al., 2010; Merlot et al., 2013), and nonhuman (Meyer and Hamel, 2014) as well as human (Griffiths and Hunter, 2014) primates. Such problems can arise when the actual adult environment does not match the predicted one; studies have found that cardiovascular disease, type 2 diabetes, metabolic syndrome, respiratory, gastrointestinal, urinary tract, dental, and mood disorders all can result from a mismatch between the predicted and actual environment the animal inhabits (Gluckman and Hanson, 2006b; Godfrey, 2006). Cognitive function too is affected by both genetic (Matzel et al., 2006) and developmental (Chwang et al., 2006) influences. Recent research suggests that one mechanism underlying the sensitization of the CTRS involves a process called epigenetic modulation of gene expression (Hunter and McEwen, 2013). Whereas genetics is the study of the genes one has, epigenetics is the study of the genes one expresses. Our interest in epigenetics developed from a serendipitous finding in cats with chronic lower urinary tract signs (called feline interstitial cystitis, FIC). During a study of the effects of a corticotrophin releasing factor antagonist on hypothalamic–pituitary–adrenal (HPA) axis function, we observed that cats with FIC we were studying had normal adrenocorticotrophic hormone release, but limited adrenocortical release of cortisol (Westropp et al., 2003). This study led to histological examination of these cats’ adrenal glands. The glands appeared normal histologically, with no evidence of hemorrhage, inflammation, infection, fibrosis, or necrosis. Morphometric evaluation, however, identified reduced size of the fasciculata and reticularis zones of the adrenal cortex. Inappropriately low plasma cortisol concentrations also have been observed in human beings with chronic pelvic pain (Raison and Miller, 2003; Buffington, 2004). The most parsimonious explanation found to date for these findings in the absence of a genetic disorder is a developmental event that results in perception of threat in a pregnant animal that was sufficiently intense that it ‘sensitized’ the CTRS of the offspring (Griffiths and Hunter, 2014; Gatchel and Neblett, 2018). Epigenetic modulation of gene expression was presciently described as the ‘epicenter of modern medicine’ 10 years ago (Feinberg, 2008). This general biological process results in such commonplace outcomes as sex- and organ-specific patterns of gene expression that lead to the final phenotype of the organism by silencing genes not appropriate to the particular tissue environment (Fig. 4.2). While the detailed molecular mechanisms of epigenetics are beyond the scope of this review, fuller explanations are available on the Internet (Farina, 2019; Wikipedia, 2019). Fig. 4.2. Epigenetic mechanisms are affected by several factors and processes during development, including environmental threats, chemicals, drugs and pharmaceuticals, aging, and diet. DNA methylation and histone modification are two of a variety of identified epigenetic mechanisms. DNA methylation occurs when methyl groups bind to promoter regions of DNA to activate or repress gene expression. Histones are proteins around which DNA can wind for compaction and control of gene expression. Histone modification occurs when the binding of epigenetic factors to histone ‘tails’ alters the extent to which DNA is wrapped around histones and the availability of genes in the DNA to be activated. All of these factors and processes can have an effect on health and disease risk (National Institutes of Health, 2019). The effects of environmental threats to the mother on fetal development seem to depend both on the timing and magnitude of exposure to products of the maternal CTRS response in relation to the activity of the developmental ‘programs’ that determine the maturation of the various body systems during gestation and early postnatal development (Gluckman and Hanson, 2005). For example, the small adrenal cortices found in some cats with FIC suggested that the event occurred during adrenocortical maturation. If the developing fetus had been exposed before initiation of adrenocortical maturation, the effect may not have been observed, whereas if it had occurred after adrenocortical maturation, adrenal size and subsequent adrenocortical responses to the CTRS might have been increased (Matthews, 2002). What influences the outcome of a fetus is not always directly related to what ‘should’ affect it. As an example, the negative effects of restricting a ewe’s caloric intake during pregnancy on a fetus or offspring is frequently understood (Ford et al., 2007). However, it has been demonstrated that excess energy intake also can have a negative influence on the offspring (Dong et al., 2008). The overfeeding is also in relation to the balance of other nutrients, so it does not occur in a vacuum. The effects of the behavior of the dam on her offspring have been demonstrated in dogs raised to be service dogs for the vision-impaired (Bray et al., 2017). By observing the behavior of the dams during their offspring’s first 3 weeks of life, testing the individuals via cognitive and temperament tests, and following up with the success, or lack thereof, of the offspring, dogs raised by dams with high maternal behavior scores had a higher chance of displaying anxiety-related behaviors and were more likely to be released from the program. The thought behind these results is that, while understood that too much stress is deleterious, too little stress, as evidenced by higher maternal care, can deny the offspring the opportunity to learn how to effectively deal with stress. Two recent reviews have expanded our understanding of early life experiences in dogs. In 2017, McMillan (2017) reviewed seven published studies and one anecdotal report of behavioral and psychological outcomes of dogs exposed to commercial breeding establishments as neonates. These studies revealed an increased incidence of behavioral and emotional problems that caused distress during adulthood compared with dogs from other sources, especially noncommercial breeders. The most consistent finding was an increase in aggression toward the dog’s owners and family members, unfamiliar people, and other dogs. Increased fear toward unfamiliar people, children, other dogs, nonsocial stimuli, and when taken on walks also was identified, as well as undesirable behaviors related to separation and/or attention seeking, and a heightened sensitivity to touch. All published studies suggested that exposure to major stressors during puppy development from the prenatal stage through adolescence was associated with the development of many behavioral (mental health) problems. Another review (Dietz et al., 2018) found similar results, concluding that, Early life experiences, such as maternal care, attachment and socialization, have long lasting and serious consequences for the behavioral and physiological development of an individual. The complex interplay between these factors is likely to have consequences for the future dog-owner bond and the vulnerability to develop behavioral disorders. Opposite results have also been revealed. Longer daily duration of maternal care during the first 3 weeks of life was associated with more exploratory behavior and less signs of stress (Guardini et al., 2016). In another study, pups that were separated from their dam at approximately 1 month of age, compared to those separated at 2 months of age, were more likely to display problem behaviors related to anxiety, such as noise reactivity and fearfulness on walks (Pierantoni et al., 2011). Sensitization is not restricted to early life, of course (Tsankova et al., 2006), but is more likely to occur during the time of growth and maturation of the neural, endocrine, and immune systems (Godfrey et al., 2010). Sometimes the ‘susceptible individual’ is revealed only by another adverse experience later in life, possibly by additional rounds of epigenetic modulation of gene expression (Daskalakis et al., 2013; Koss and Gunnar, 2018). Subsequent rounds of changes in gene expression may be quite stable and resistant to current medical interventions. Thus, confining ‘sensitive’ individuals in ‘provocative’ environments can have particularly strong implications for their mental and physical health (Buffington et al., 2014). The effects of adverse events, particularly during the vulnerable period of early life, on the long-term mental and physical health of animals demonstrate the importance of identification of risk factors and provision of effective education of owners of companion animals by primary care veterinarians about appropriate environments for confined animals across the lifespan. From this perspective, initial vaccination appointments become anything but ‘routine’, and may in fact be the most important appointments of the pet animal’s life. This is because such visits present opportunities to teach husbandry appropriate for the animal based on the environment it is confined to at a time when owners are likely to be most motivated and responsive to recommendations. Shonkoff (2010) described three foundational domains to promote long-term health and welfare that can be adapted to preventive veterinary medical care. These include: 1. A stable and responsive environment which provides consistent, nurturing, and protective interactions that enhance learning and support development of adaptive capacities that promote a well-regulated CTRS. 2. A safe and supportive physical, chemical, and built environment that is free from toxins and other threats, allows active exploration without significant risk of harm, and offers support for families raising the animal. 3. Satisfactory nutrition for the animal’s age, including feeding management practices that maintain an appropriate body condition, beginning with the future mother prior to conception if possible. (See also Chapter 10.) Consideration of DOHaD also has important therapeutic implications. For example, drugs to modulate gene expression are under active investigation in oncology (Bai et al., 2018) and psychiatry (Mahgoub and Monteggia, 2013; Robinson, 2018) that may become available in veterinary medicine. Given that these drugs also may modulate expression of other genes in potentially unpredictable ways, the availability of a naturally occurring animal model of diseases likely influenced by these mechanisms offers the opportunity to ‘field-test’ these compounds before they are introduced for use in human beings (Buffington, 2009). Environments also are known to epigenetically modulate gene expression through ‘endogenous pharmacology’ (Sale et al., 2014; Eisinger and Zhao, 2018; Tai et al., 2018), which may explain the value of behavior-based approaches to treatment in humans (Kohrt et al., 2015), and effective environmental enrichment in animals (Francis et al., 2002). In summary, the changing view of medicine from one of an isolated organ-originating disease in otherwise healthy adults to one of an underlying DOHaD perspective has important implications. First, it provides a more parsimonious explanation for many findings that previously were quite difficult to account for, including the unfortunate lack of beneficial effect of therapies directed at the peripheral organ of interest by a particular medical subspecialty. It also helps explain the presence of multiple comorbid disorders in many patients with chronic disorders but not in patients with other individual organ diseases, the unpredictable order of appearance of the comorbidities, and the altered functioning of the CTRS. More importantly, it opens new approaches to therapy that may escape consideration from the individual medical specialty perspective. The available data only suggest this perspective, however, and permit generation of testable hypotheses. One might imagine a number of additional complementary or alternative ‘systemic’ hypotheses related to variable combinations of genetic, epigenetic, and environmental influences; these remain to be explored. The impact of environment on clinical signs was dramatically demonstrated during research into the use of cats with FIC as a model for a chronic pelvic pain syndrome in humans called interstitial cystitis (Buffington, 2004). Two important initial observations were: (i) many of the cats had a variety of comorbid disorders, which had often preceded the onset of the lower urinary tract signs; and (ii) all of the signs essentially resolved within 6 months of housing in the environmentally enriched vivarium. After observing the rather remarkable recovery of the cats donated to the investigator’s research colony, Dr. Judi Stella discovered that a variety of ‘sickness behaviors’ occurred in some of the cats, both those with FIC and healthy controls, after exposure to ‘unusual external events’ (Stella et al., 2011). Sickness behaviors are variable combinations of nonspecific clinical and behavioral signs that include diarrhea, vomiting, fever, lethargy, somnolence, and decreased general activity, body-care activities (grooming), social interactions, and food and water intake (Dantzer et al., 2008). Sickness behaviors are thought to reflect a change in motivation of the organism to one that promotes recovery by inhibiting metabolically expensive activities (e.g., foraging, reproducing) and favoring those that contribute to recovery (e.g., reduced activity, hiding). These behaviors are well-documented physiologic and behavioral responses to injury and infection found in all animal species studied (Hart, 1985, 1988). Sickness behaviors also can occur in response to aversive environmental events (Marques-Deak et al., 2005; Reed and Raison, 2016), through CTRS-mediated activation of inflammatory pathways in peripheral blood mononuclear cells (Bierhaus et al., 2003; Bierhaus, 2006). Bierhaus et al. (2003) found that subjecting human volunteers to a laboratory stress test resulted in rapid increases in circulating concentrations of catecholamines, cortisol, and the transcription factor nuclear factor kappa beta (NF-κB). In follow-up studies in mice, they found that the increase in NF-κB response could be reduced in the presence of the α1-adrenergic inhibitor prazosin. Finally, they demonstrated in cultured cells that only norepinephrine induced NF-κB and NF-κB-dependent gene expression, which was reduced by both α1- and ß-adrenergic inhibitors. Stimulation of NF-κB-dependent gene expression by perceived threat thus results in increases in circulating concentrations of pro-inflammatory cytokines just as infection and injury do. Miller and Raison (2016) recently speculated that the behavioral responses, such as avoidance and alarm, that are likely to have provided early mammals with an evolutionary advantage in their interactions with pathogens and predators may have become adapted to perceived psychosocial threats as well. They proposed that early evolutionary pressures from interactions with pathogens, predators, and conspecifics resulted in an inflammatory bias that included an integrated suite of immunological and behavioral responses that conserved energy for fighting infection and healing wounds while maintaining vigilance against attack. They believe that this inflammatory bias was held in check during much of evolution by exposure to minimally pathogenic, tolerogenic organisms in ancient environments that engendered immunological responses characterized by the induction of regulatory T and B cells, immunoregulatory macrophages, and production of anti-inflammatory cytokines (Raison and Miller, 2013). In cats with FIC, for example, Stella et al. (2011) recorded sickness behaviors referable to the gastrointestinal and urinary tracts, the skin, and behavior problems for 77 weeks. ‘Unusual external events’, e.g., changes in caretakers, vivarium routine, and lack of interaction with the investigator were identified during 11 of the 77 weeks. No instances were identified during the remaining 66 weeks, which were considered control weeks. They found that exposure to these events (especially of older cats), but not disease status, significantly increased total number of sickness behaviors when results were controlled for other factors. Older cats in both groups were at a slightly greater relative risk for upper gastrointestinal tract signs (1.2-fold, P < 0.001) and avoidance behavior (1.7-fold, P = 0.001). Exposure to unusual environmental events also significantly increased the relative risk for decreased food intake (9.3-fold, P < 0.001), for no eliminations in 24 hours (6.4-fold, P < 0.001), and for defecation (9.8-fold, P < 0.001) and urination (1.6-fold, P = 0.005) outside the litter box. Thus, some of the most commonly observed abnormalities in client-owned cats, including upper gastrointestinal signs, house-soiling, and inappetence, were observed after exposure to unusual environmental events in both healthy cats and cats with FIC. In addition to inflammation, environmental threats can also induce oxidative stress through activation of the CTRS. Recent studies have provided evidence that mitochondria, subcellular organelles that engage both in ATP production and intracellular signaling, can be both targets of the CTRS as well as mediators of threat pathophysiology (Picard and McEwen, 2018a,b). Mitochondria can respond to a variety of the products of the CTRS, including norepinephrine (Zhang et al., 2017), glucocorticoids, estrogen, angiotensin, and cannabinoids (Picard and McEwen, 2018a). In response, mitochondrial energy production is reduced, production of reactive oxygen species and cytokines increases, and gene expression in the nucleus is affected through epigenetic modulation. A recent study provided an example of the effects of CTRS-induced autonomic dysregulation of mitochondrial function in the rat urothelium (Kullmann et al., 2018). In this study, adult female Wistar Kyoto rats were exposed to 10 days of water avoidance stress, after which bladders were harvested for Western blot and single cell imaging in culture. Mitochondria in urothelial cells from stressed rats were ∼30% more depolarized compared to those from control rats. In addition, expression of the fusion protein mitofusion-2 was upregulated, suggesting mitochondrial structural changes consistent with altered cellular metabolism, and intracellular calcium concentrations were increased, consistent with impaired cellular function. Stimulating the cultured urothelial cells with α-adrenergic receptor agonists increased reactive oxidative species production, suggesting a direct catecholamine action on the cells; adrenergic receptor antagonists prevented most of stress-induced changes. Resilience, the animal’s ability to resist the deleterious effects of exposure to chronic threats, is a particularly impactful factor for mental health (Russo et al., 2012; Colditz and Hine, 2016). In fact, based on research in humans (where the concept has been most commonly studied), resilience is the rule rather than the exception. More than two-thirds of the general population experiences a significant traumatic event at some time in their lives, and as many as 20% of United States residents may experience one in any given year (Galea et al., 2005). Despite this high frequency of exposure, the prevalence of pathology resulting from the experience in the general population has been estimated to be only 5–10%, depending on the type, intensity, and duration of the exposure (Norris et al., 2002; see also Chapter 14). The mechanisms underlying resilience remain incompletely known, but likely involve variable combinations of passive (genetic) and active (environmental) CTRS-associated differences that confer increased resistance to the pathological effects of exposure to chronic threat (Russo et al., 2012). Studies in humans and rodents have found some differences in genes underlying CTRS responsiveness, although the associations are relatively weak. In contrast, it has long been known (Levine, 1962) that neonatal rats exposed to intermittent foot shocks subsequently respond more effectively when confronted with novel situations compared to their nonstressed counterparts, a phenomenon known as ‘stress inoculation’. A body of research developed since this seminal study has found that exposure to moderate degrees of threat during early life, adolescence, and adulthood can reduce vulnerability to threat and broaden the range of tolerable threat for animals (Bock et al., 2014; Guardini et al., 2016; McEwen, 2017a; see also Chapter 14). Epigenetic modulation of gene expression related to early life experiences also appears to play a role in this ‘inoculation’. For example, recent studies (Roth, 2013; Köhler et al., 2018) have reported that epigenetic changes in dopamine function in the hippocampus might modulate the outcome of adverse early life exposure. In Köhler et al.’s study, mouse pups were separated from their mothers for 3 hours from either 14–16 days of age (short-term) or from 1–21 days (long-term), after which they subjected the mice to a forced swim test (threat). They found that short- and long-term separation of the pups from their mothers resulted in changes in dopaminergic molecular pathways, some of which were epigenetically regulated and which either increased (long-term) or decreased (long-term) depressive-like behaviors later in life. Future studies will likely reveal even greater complexity as more circuits and mechanisms are uncovered. Neural effects of early intermittent separation also include increases in cortical volume and synaptic function, decreased glutamatergic tone in the nucleus accumbens, and normalization of neuronal excitability in resilient animals. Strategies like environmental enrichment can confer resilience by normalizing HPA-axis function (Russo et al., 2012). Resilience also can affect immune function, and vice versa (Dantzer et al., 2018). Interested readers are referred to the cited references for more detail. The state of activation of the CTRS can be evaluated by assessing physiological (Table 4.1) and behavioral (Table 4.2) parameters. Animals differ in their responses to threat, and not all animals display all possible signs of threat. Moreover, both increased (e.g., defensive aggression) and decreased (e.g., freezing) activity can be associated with activation of the CTRS. Thus, a thorough assessment of all parameters is most likely to provide the best estimator of CTRS activity in any given animal in any particular context.
4.1 Introduction
4.2 Mental and Physical Health
4.2.1 Definitions
4.2.2 Genetic influences
4.2.3 Early life and epigenetic influences
4.2.4 Environmental influences
4.3 Resilience
4.4 Evaluating Mental and Physical Health
Parameter
Normal
Threatened
Temperature
No change
May be increased
Heart rate
Blood pressure
Respiratory rate
Pupil diameter
‘Sweaty’ paws
Absent
May be present
Excessive shedding
Flushing
Apprehensive lip licking
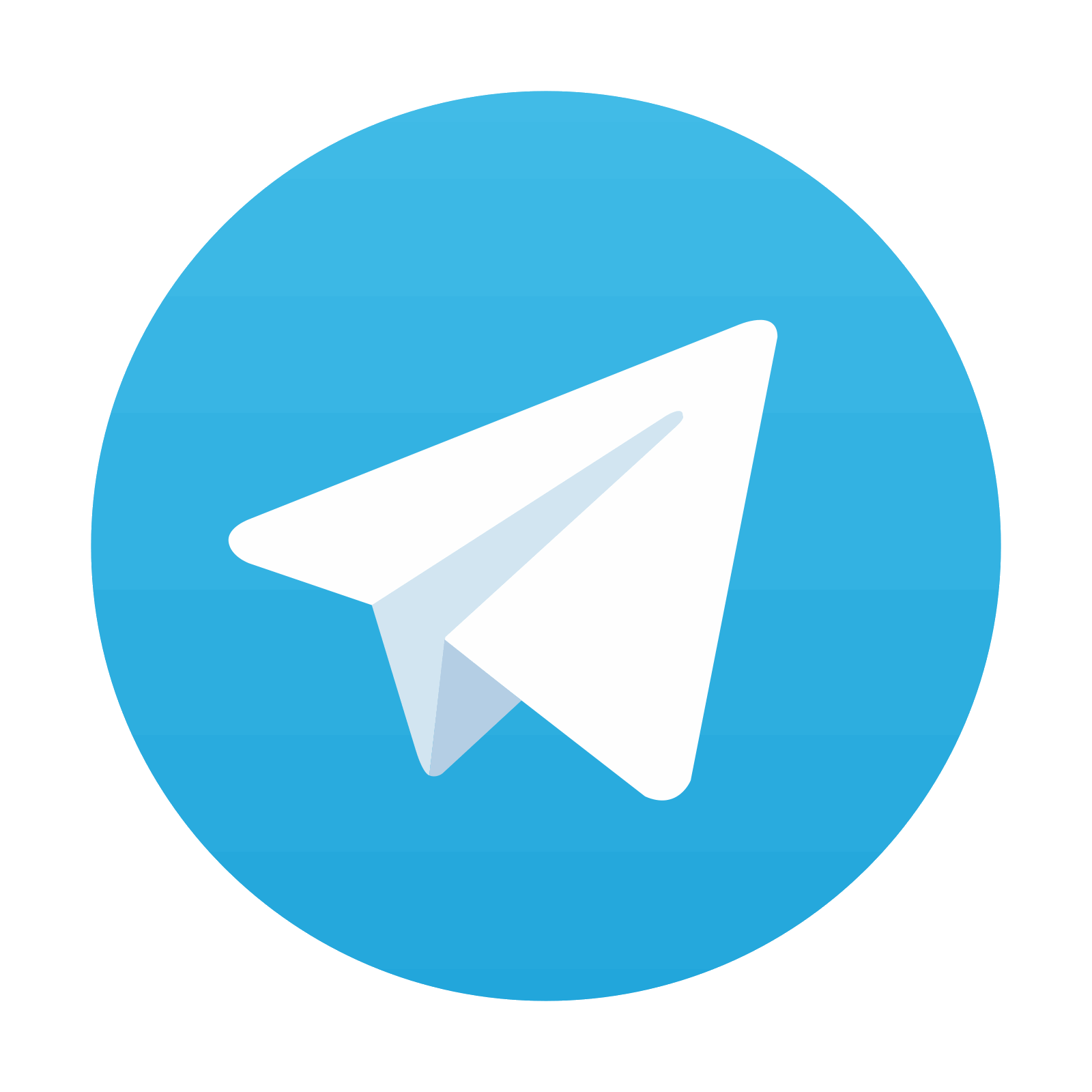
Stay updated, free articles. Join our Telegram channel
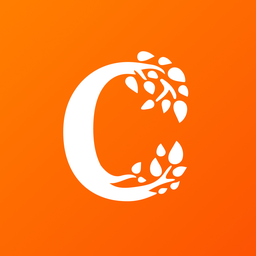
Full access? Get Clinical Tree
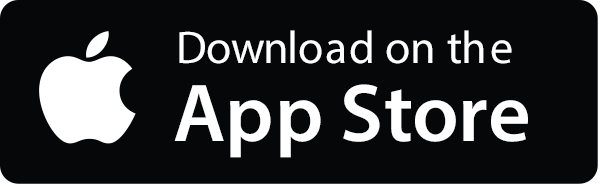
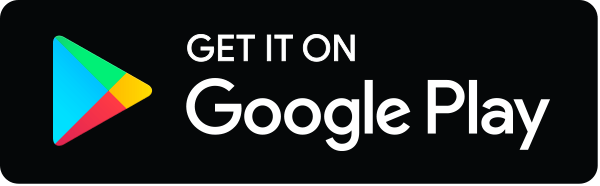