Chapter 15 DISEASES AND INJURIES OF THE APPENDICULAR SKELETON The distal (third) phalanx (P3, coffin bone) The middle phalanx (second phalanx, short pastern bone, P2) The proximal phalanx (first phalanx, long pastern bone, P1) The third metacarpal/metatarsal (cannon) bone Dorsal cortical disease (bucked shins and dorsal cortical fractures) Palmar/plantar cortical disease (incomplete palmar cortical fractures and avulsion fractures at the origin of the suspensory ligament) The second and fourth metacarpal/metatarsal (splint) bones Exostosis of the caudal perimeter of the radial physis (physeal remnant spikes) Enostosis-like lesions (bone islands) Traumatic periosteitis of the craniodistal surface The calcaneus (fibular tarsal bone) ARTHROLOGY (DISEASES OF JOINTS) Osteoarthritis/degenerative joint disease Lameness directly associated with trimming and shoeing Quicking (nail prick, hot nail) Over-lowering of the hoof wall (excessive trimming) Sudden changes in hoof length and/or balance Conditions of the hoof capsule Conditions of the deeper structures of the foot Fractures of the distal phalanx Fractures of the navicular bone Osteoarthritis of the distal interphalangeal joint (low ringbone, degenerative joint disease) Desmitis of the collateral ligaments of the distal interphalangeal joint Pyramidal disease (buttress foot) Phalangeal exostosis (low ringbone) Navicular syndrome (navicular disease, caudal heel syndrome, caudal heel pain) Ossification of the collateral cartilages of the distal phalanx (sidebone) SOFT TISSUE INJURIES AND DISEASES Diseases of the suspensory apparatus Superficial digital flexor tendinitis in the metacarpal/metatarsal region Superficial digital flexor tendinitis in the pastern region Deep digital flexor tendinitis Accessory ligament of the deep digital flexor tendon (inferior check ligament) desmitis Palmar/plantar annular ligament desmitis Suspensory ligament desmitis—body and branch lesions Suspensory ligament desmitis—proximal Complete breakdown of the suspensory apparatus Miscellaneous tendon and ligament injuries Diseases and injuries of articular soft tissue structures Tendon lacerations and ruptures Suspensory apparatus lacerations Superficial digital flexor tendon rupture Deep digital flexor tendon rupture Common digital extensor tendon rupture Extensor carpi radialis tendon rupture Digital flexor tendon sheath tenosynovitis Palmar/plantar annular ligament syndrome (annular ligament constriction, stenosing palmar ligament desmitis) Tarsal sheath distension (thoroughpin) Extensor carpi radialis, common digital extensor and lateral digital extensor tenosynovitis Intertubercular (bicipital) bursitis Calcaneal bursitis (capped hock) Olecranon bursitis (shoe boil, capped elbow) Supraspinous bursa infection (fistulous withers) Trochanteric bursitis (whorlbone disease) Fibrotic or ossifying myopathy Post-anesthetic myopathy and neuropathy Recurrent exertional rhabdomyolysis Polysaccharide storage myopathy Nutritional myodegeneration (nutritional myopathy, nutritional muscular dystrophy, white muscle disease) Hyperkalemic periodic paralysis Peroneus (fibularis) tertius—rupture or avulsion of the origin Suprascapular nerve injury (sweeny) DEVELOPMENTAL ORTHOPEDIC DISEASE The importance of diagnostic local analgesia in the investigation of equine lameness has been well documented. Typically this involves perineural or regional analgesia and intrasynovial analgesia although epidural analgesic techniques (q.v.) are frequently used for treatment of orthopedic pain. The three most suitable local analgesic agents are: A new vial of local anesthetic should be used for intrasynovial analgesic administration. Details of forelimb and hindlimb regional and intrasynovial analgesia are given in Table 15.1. Table 15.1 Techniques for diagnostic local anesthesia DDFT, deep digital flexor tendon; SDFT, superficial digital flexor tendon. 1All volumes of local anesthetic agent are for 2% mepivacaine hydrochloride. 2Alternative methods of intrasynovial anesthesia of the forelimb and hindlimb have been described in the literature for many of these sites. 3More than five different approaches to the navicular bursa have been described and compared in the literature. 4Lateral and palmar approaches to the distal interphalangeal joint have also been described. 5Dorsal midline and palmaroproximal injection sites have also been described. 6A dorsal approach to the metacarpophalangeal joint is often utilized to distend the joint prior to arthroscopic surgery, and can be used diagnostically as well. 7An alternative approach made axial to the midbody of the proximal sesamoid bone through the annular ligament with the fetlock joint flexed is particularly valuable when the sheath is not distended and is difficult to palpate proximal or distal to the annular ligament. 8A number of modifications of these approaches to the cubital joint have been reported. 9The femoropatellar joint can also be approached laterally. 10Alternative approaches to the medial and lateral femorotibial joints have been described. Four major functions of bone can be identified. The tissue provides: 1. The supportive and protective framework of the body 2. The levers by which muscles effect locomotion 3. Reservoirs of inorganic elements and fat Vascularity of bone is maintained through a medullary and periosteal blood supply, and the blood supply to bone differs between mature and immature states. Generally the blood supply to immature bone is more extensive. In developing bones with active growth plates the epiphysis and metaphysis have separate supplies because most vessels do not traverse the cartilaginous plate. There are transphyseal vessels in large epiphyses. The epiphysis is supplied by a network of vessels entering at articular margins circumferentially—an arrangement particularly vulnerable to trauma. The metaphyseal side of the growth plate is supplied by a similar arborization of vessels entering via numerous foramina. These anastomose with branches of the nutrient artery before coursing perpendicular to the active growth plate. Periosteal vessels supply the outer third of forming cortical bone of the diaphysis. In mature bone significant periosteal supply persists only at sites of firm fibrous attachments. 1. Parathyroid hormone (PTH) is secreted by the parathyroid glands from cells highly sensitive to the calcium concentration in blood. PTH (q.v.) is the principal hormone regulating plasma calcium levels. Its action on bone is to increase resorption thus elevating blood calcium. 2. Calcitonin is secreted by C cells of the thyroid gland, which are also sensitive to plasma calcium concentration. Calcitonin (q.v.) is inhibitory to PTH and disables osteoclasts, therefore acting to decrease blood calcium levels. 3. Cholecalciferol (vitamin D3) is both ingested and, catalyzed by ultraviolet radiation, synthesized in the epidermis. The principal purpose of vitamin D3 (q.v.) is to provide sufficient extracellular calcium and phosphorus for mineralization to take place, and its chief role is increasing intestinal absorption of these. Grooves and prominences on the outer surfaces of bones develop in adaptation to the pressure from overlying muscle bellies or tendons and the pull from soft tissue attachments, respectively. Mature periosteum can be reactivated by increased vascularity to form new bone at, or close to, sites of injury. Subchondral bone is also subject to remodeling in the face of repetitive functional overloading. This reduces its compressibility, undermining its ability to absorb shock, and so contributes to the vicious circle of osteoarthritis (q.v.).
The musculoskeletal system
INTRODUCTION
DIAGNOSTIC LOCAL ANALGESIA
PRACTICAL ASPECTS OF DIAGNOSTIC LOCAL ANESTHESIA
Individual techniques
OSTEOLOGY
STRUCTURE AND FUNCTION OF BONE
MODELING AND REMODELING OF BONE
The musculoskeletal system
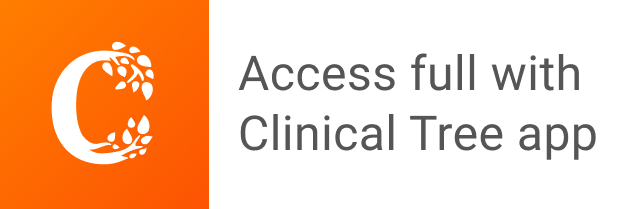