Fig. 4.1.
Risk genes and environmental factors such as hypoxia play a role in the pathophysiology of schizophrenia during the perinatal period, whereas symptoms occur in young adulthood after the synaptic pruning process and wiring of the prefrontal cortex. In some patients with a chronic disease, further neurodegenerative changes with volume loss in several brain regions may play an additional role.
In the early postnatal period, the developing rat brain is highly vulnerable to hypoxic damage (9). In contrast to humans, important steps of brain development in rats occur mainly postnatally and the brain growth spurt of early postnatal rats is comparable to the third trimester in humans (10). At this stage, overexpression of NMDA receptors has been observed reaching peak levels at postnatal day 8 (11–13). This resembles the peak in NMDA receptor binding and mRNA expression in gestational stages in the human fetus (14, 15). The tetrameric receptor is composed of several distinct subunits, the obligatory NR1 and several facultative NR2 subunits (NR2A, NR2B, NR2C, and NR2D), responsible for different functional properties (16, 17). During postnatal development, NMDA receptors are composed of more NR2B than NR2A subunits and the NR2D subunit is most prominent around postnatal day 7 (17). NMDA receptors assembled from NR2B or NR2D subunits show higher affinity for the co-agonist glycine than NMDA receptors with more NR2A subunits in the adult brain. They are also less prone to blockade by magnesium than more mature channels (13). During brain development, NMDA receptors are involved in synaptic plasticity, as well as migration of neurons to their final destination (18–22). In this respect, the early postnatal period of rats is most suitable for investigating the role of hypoxia in the pathophysiology of schizophrenia, as it represents a highly vulnerable time period in brain development.
In schizophrenia, NMDA receptor hypofunction has been hypothesized, but the etiology of this dysfunction remains elusive. An early developmental event has been proposed to be involved in the process leading to NMDA receptor hypofunction (23). Hypoxia may be part of this course of events and is investigated in the present animal model. This may be additive with genetic factors, many of which involve the area of glutamatergic transmission (24). We have shown that postnatal, repeated hypoxia can precipitate alterations in NMDA receptor binding and gene expression of subunits early after the event and in adulthood. Indeed, in our animal model of chronic neonatal hypoxia, rats at PD 120 exhibited – along with the PPI deficit – increased gene expression of the N-methyl-d-aspartate (NMDA) receptor subunit NR1 in hippocampal, frontal, and temporal subregions as well as in nucleus accumbens (25), which suggests an involvement of the NMDA receptor in the pathophysiology of hypoxia-induced alterations.
The pathogenetic implications of obstetric complications are unclear. One hypothesis suggests that obstetric complications are caused by neurodevelopmental pathology (26–28), another speculates that obstetric complications itself cause developmental neuropathology (26, 29). Either argument is consistent with the neurodevelopmental hypothesis of schizophrenia, which suggests that abnormal brain development and neuropathology occur perinatally, whereas symptoms of the disease appear in early adulthood (8). Thus, if a common factor of obstetric complications such as perinatal hypoxia causes neurodevelopmental disturbances, an animal model of neonatal hypoxia should express schizophrenia-related behavioral alterations in early adulthood of the affected rats. Schizophrenia patients show deficits in attention and processing of sensory information. A reliable model of sensorimotor gating in vertebrates is prepulse inhibition (PPI) of the acoustic startle response, which is disrupted in schizophrenia patients and restored by antipsychotics, mainly clozapine (30). In rats, PPI also reflects sensorimotor gating, and several brain regions such as hippocampus, prefrontal cortex, nucleus accumbens, and striatum are involved in PPI-modulating circuitry (31). In a neurodevelopmental animal model in rats, neonatal hippocampal lesion led to decreased PPI, increased locomotor activity after amphetamine challenge, and disturbed social interaction in early adulthood (32), while medial prefrontal lesions led to attenuated locomotor response (33). Additional animal models would be important to further elucidate possible roles of neurodevelopmental factors in the pathogenesis of schizophrenia. Thus, the present approach is meant to mirror the impact of chronic hypoxia during human obstetric and birth complications in more detail.
Exposure to anoxia at postnatal day (PD) 9 showed no effects on the PPI paradigm in adulthood (34). However, it must be kept in mind that the duration of the anoxia period of the study be kept relatively short, and long-term hypoxia may indeed affect behavior in adulthood. In a recent study applying chronic neonatal hypoxia, rats at PD 120 exhibited a PPI deficit and increased gene expression of the N-methyl-d-aspartate (NMDA) receptor subunit NR1 at PD 11 and 120 in hippocampal, frontal, and temporal subregions as well as in nucleus accumbens (25). This points to the involvement of the NMDA receptor in the pathophysiology of hypoxia-induced alterations. In another study, Hermans and Longo (35) exposed rat pups to nearly the same oxygen concentrations for 6 h/day and showed increased rearing activity and stereotypies in hypoxia-treated animals. However, in our study, hypoxia-treated rats have been closely examined from the pre- and postpubertal phase until adulthood and effects of antipsychotic treatment have been examined. Rats exposed to hypoxia exhibited deficits in locomotor activity on PD 86, 120, and 150, as well as a PPI deficit on PD 120 and 150 in adulthood, but not before. Chronic treatment with clozapine reversed hypoxia-induced PPI deficits, but not the decreased locomotor activity. In a second experiment, where clozapine was chronically administered before PD 120, development of the PPI deficit in the animals exposed to hypoxia was prevented (36).
Here we show that schizophrenia-related behavioral alterations occur in early adulthood of the affected rats. Schizophrenic patients have deficits in processing of sensory information and attention. A reliable model of sensorimotor gating is prepulse inhibition of acoustic startle response (PPI), which is disrupted in schizophrenic patients and restored by antipsychotics (30, 37). In a neurodevelopmental animal model in rats, postnatal hippocampal lesion led to decreased PPI in early adulthood (38). Additional animal models would be important to further elucidate possible roles of neurodevelopmental factors in the pathogenesis of schizophrenia. Thus, our approach is meant to mirror in more detail the impact of chronic hypoxia during human obstetric and birth complications.
In schizophrenia, there is strong support for disturbances of the glutamatergic and GABAergic microcircuitry, mainly affecting the expression of presynaptic vesicle proteins including SNAP-25 and syntaxin, which form the SNARE complex (39–48). The trimeric SNARE complex consists of the vesicle protein synaptobrevin (VAMP) and the plasma membrane proteins syntaxin and SNAP-25, which drive membrane fusion by guiding the vesicle and plasma membrane proteins in close proximity, thus overcoming the energy barrier for fusion. Complexin I (in inhibitory and excitatory synapses) and complexin II (in excitatory synapses) operate at a post-priming step in synaptic vesicle exocytosis by stabilizing the SNARE complex (49). Neuropeptide Y and neurexins play a role in cell adhesion and are hypothesized to play a role in the pathophysiology of schizophrenia (45, 50–52).
Little is known about the influence of chronic hypoxia on the expression of those and other presynaptic proteins. Snails exposed to hypoxia showed a decreased expression of syntaxin 1 (53), but long-term effects of perinatal hypoxia on expression of these genes in mammals and their contribution to behavioral deficits in adulthood are unknown. Additionally, there is only sparse information about the influence of antipsychotic treatment on gene expression, as has been shown for neuropeptide Y and complexins in normal rats (54, 55). In our last study, we showed that in several brain regions, presynaptic genes such as SNAP-25, syntaxin 1A, neurexin, neuropeptide Y, and complexin I were downregulated and the NR1 subunit of the NMDA receptor was upregulated by hypoxia. These differential gene regulations could be partially compensated for by clozapine treatment (56). Differential gene expression in cortical and subcortical brain regions as well as correlations to deficits of PPI support the view of an involvement of synapse-associated gene products and glutamatergic and GABAergic neurotransmission in the pathophysiology of behavioral deficits occurring as delayed responses to neonatal hypoxia in adulthood.
2 Materials
1.
Air-tight hypoxia chamber
2.
Oxymeter (GMH3690, Conrad, Hirschau, Germany)
3.
Startle device (for example, TSE systems, Germany, or San Diego Instruments, USA)
4.
Arena (ActiMot, TSE Systems, Bad Homburg, Germany)
5.
Rat brain atlas (Paxinos and Watson (63))
6.
Cryostat (Leica)
7.
Image analysis system (Inter Active Systems, Interfocus, Germany)
8.
[3H]-sensitive film (Hyperfilm®, Amersham, Germany)
9.
[3H] plastic standards (Amersham, Germany)
10.
35S-sensitive X-ray films (Biomax MR1)
11.
14C plastic standards (Amersham, Germany)
12.
Clozapine (Novartis, Switzerland)
13.
RNA extraction kit (RNeasy, Qiagen, Germany)
14.
Brain Punch Set (Föhr Medical Instruments, Germany)
15.
Agilent 2100 Bioanalyzer
16.
Packard ScanArray 5000 scanner
17.
Fast Real-Time PCR cycler (Applied Biosystems, Foster City, CA, USA)
3 Methods
3.1 Animals
A total of 116 Sprague Dawley rats from 12 different litters (bred from 12 females and 5 males; Animal Facility, Department of Animal Physiology, University of Tübingen) were used for the experiments. The rats were housed in groups of three to four animals under a 12 h light/dark cycle (light on at 7 a.m.) with food and drinking water available ad libitum. The experiments were carried out in accordance with the international ethical guidelines for the care and use of laboratory animals for experiments (Declaration of Helsinki, NIH guidelines) and were approved by the local animal care committee (Regierungspräsidium Tübingen, ZP 3/02).
3.1.1 Neonatal Hypoxia
From postnatal day (PD) 4–8, we imposed mild chronic hypoxia (11% O2, 89% N2) on six litters (n = 64) and their mothers by placing them in an air-tight plastic chamber (31 × 40 × 15 cm3) for a period of 6 h/day (Fig. 4.2). The six other litters (n = 52) were subjected to identical handling conditions but were placed in identical chambers with regular oxygen concentrations (21% O2 = normoxia). In both chambers, the oxygen concentration was controlled by an oxygen measuring device (GMH3690, Conrad, Hirschau, Germany). During hypoxia, mothers were able to care for their pups. Unsystematic observations revealed no obvious effects of hypoxia on maternal behavior during hypoxia or normoxia.
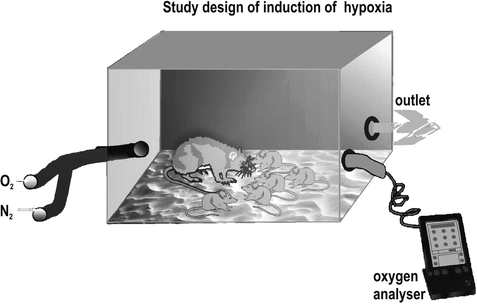
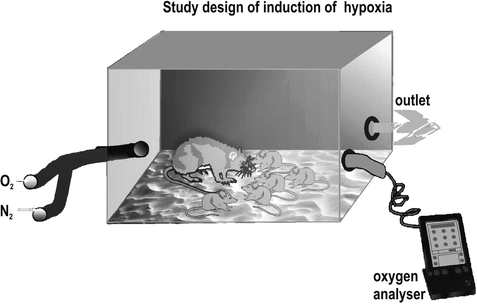
Fig. 4.2.
Air-tight hypoxia chamber with oxymeter and separate oxygen and nitrogen inflow.
3.1.2 Cross-Fostering
To control for possible effects of hypoxia on maternal behavior, the offspring of the four litters in experiment 1 (n = 62) were cross-fostered on PD 9, meaning that half of the offspring of a hypoxia-treated litter were exchanged with half of the offspring of a normoxia-treated litter. All mothers accepted the unknown offspring without problems. At an age of 4–5 weeks, the young rats were separated from the mother or nurse, respectively, and were housed in groups of the same gender under the conditions described above.
3.2 Testing Procedure
Behavioral tests were carried out at three or four different age levels: between PD 34 and 38, between PD 84 and 88, between PD 118 and 122, and between PD 148 and 152 (only experiment 1). For practical purposes, these test phases are named PD 36, PD 86, PD 120, and PD 150 throughout the manuscript. During each test phase (lasting 1 week), every rat was tested first on motor activity in an open field (day 1 or 2 of the test week), second on social interaction and social recognition (day 3 or 4), and third on baseline startle magnitude and prepulse inhibition (day 4 or 5, for details see below). All behavioral tests were carried out in the light phase between 9 a.m. and 4 p.m. During the last two test phases (experiment 1: between PD 120 and PD 150, experiment 2: between PD 86 and PD 120), half of the rats were being treated over a period of 4 weeks with ca. 45 mg/kg/day clozapine (Novartis Pharma AG, Basel, Switzerland) diluted in some drops of HCl in the drinking water adjusted to pH 6.5. Control rats received some drops of HCl in the drinking water to match the pH levels of the drug solutions. The drug dosage was calculated by the amount of ingested water and body weight, which was measured every day and the dose of 45 mg/kg/day is an estimate of the average daily intake over the whole period of administration. This route of administration leads to clozapine plasma levels of ca. 22 ng/ml (57), a level which is shown to be pharmacologically active (57). Furthermore, clozapine, using the same doses and the same route of administration, has been shown to alter the glutamatergic and GABAergic system in rat brain (58–61).
3.2.1 Test on Motor Activity
Spontaneous motor activity was tested in a square arena with walls made from transparent Plexiglass (92 × 92 × 39 cm; ActiMot, TSE Systems, Bad Homburg, Germany) where animals were placed over a 10 min period. Illumination of the arena was between 200 and 250 lux. Rat movements were monitored by infrared detectors (distance between two detectors: 2.5 cm and height: 2.5 cm) and rearings were detected by a second arrangement of infrared detectors (height: 12.5 cm). The ActiMot software automatically calculated motor activity (distance traveled in meters), number of rearings, and total time spent in the middle of the arena (size of the middle: 46 cm × 46 cm).
3.2.2 Test on Social Interaction and Social Recognition
Juvenile rats, ca. 30 days old, were used as a social stimulus. Both the test rats and the social stimulus rats were housed in isolation for 1 h before testing. For testing on social interaction (62), both groups of rats were placed in a cage (38 cm × 22 cm × 15 cm) where their behavior could be observed via camera and monitor. The duration of all social behavior (anogenital sniffing, social sniffing, social grooming, play fights) of our test rats within a 5 min period was recorded by an observer who was not aware of the group (anoxia/normoxia, clozapine treatment) to which the observed animal belonged. The animals were then separated and kept in isolation for a period of 30 min. Thereafter, a second test on social interaction between these two rats was carried out. Test values for social recognition were expressed as the ratio of the time spent on social interaction during the second test divided by the time spent on social interaction during the first test.
3.2.3 Test on Baseline Startle Magnitude and Prepulse Inhibition
The startle magnitude was measured with four identical acoustic startle devices in sound-attenuated chambers. For the tests, rats were placed in wire mesh cages with steel floors (20 × 10 × 12 cm3) which were fixed onto piezoelectric accelerometers. Movements of the rats caused changes in the voltage output of the accelerometers. These voltage changes were amplified, digitized, and analyzed by a PC. The startle magnitude was computed as the difference of the accelerometer output 80 ms before and 80 ms after the onset of the startling stimulus. Acoustic stimuli were generated by a function synthesizer (Hortmann, Germany) and delivered through loudspeakers mounted 40 cm away from the test cages. Intensity was measured using a 0.5 in. condenser microphone and a measuring amplifier (Brüel and Kjaer, Denmark) after bandpass (0.25–80 kHz) filtering.
After a 5 min adaptation period, during which the rats received no stimuli except the background noise, the startle test session began with five initial startle stimuli (100 dB SPL broadband noise pulse, 20 ms duration) followed by four different trial types presented in a pseudorandom order: (1) Startle pulse alone (100 dB sound pressure level (SPL) broadband noise pulse, 20 ms duration), (2) 75 dB prepulse (75 dB SPL, 10 kHz tone pulse, 20 ms duration including 0.4 ms rise/fall times) followed by a startle pulse 100 ms after prepulse onset, (3) 75 dB prepulse alone, and (4) no stimulus. Background noise intensity was 55 dB SPL. A total of five presentations of each trial type was given with an interstimulus interval of 20 s. PPI was measured as the difference between the pulse-alone trials and the prepulse–pulse trials and expressed as percent PPI [100 × (mean ASR amplitude on pulse-alone trials – mean ASR amplitude on prepulse–pulse trials)/mean ASR amplitude on pulse-alone trials].
Experiment 2. The aim of experiment 2 was to test whether chronic clozapine treatment before the onset of the behavioral symptoms induced by neonatal hypoxia (PD 120) prevents the development of these symptoms. To minimize factors, the animals of this experiment (n = 54) were not cross-fostered after neonatal hypoxia or normoxia (experiment 1 showed that this factor has no influence on the hypoxia effect) and only male rats were used. Behavioral testing was identical to experiment 1, except that our tests on motor activity were carried out in a cage (55 cm × 32 cm × 19 cm) with one infrared sensor delivering an arbitrary measure of motor activity (“counts”) being used. Unfortunately, this setup did not allow us to measure rearing behavior.
3.3 Brain Investigations
Brains were removed, frozen in liquid nitrogen-cooled isopentane at –80°C, and stored for 3 months. For cryostat sections, brains were processed at –20°C. Deep-frozen brains were brought to –12°C the day before cutting. Cryostat temperature was maintained at –16°C. Adjacent coronal 15 μm sections (receptor autoradiography) and 20 μm sections (in situ hybridization) were thaw-mounted on superfrost plus microscopic slides (Roth, Germany), dried, and stored at –20°C for less than 5 days (receptor binding). Sections for in situ hybridization were fixed in 4% paraformaldehyde, dehydrated in ethanol, and stored at –20°C for several weeks. For the microarray experiments, slices of 120 μm thickness were used to excise respective brain regions using a micro-punching tool. The resultant cylindrical tissue blocks were collected in –78°C test tubes (on dry ice) and stored at –80°C until further processing.
Brain regions were delineated according to the rat brain atlas of Paxinos and Watson (63). We investigated the frontal (motor cortex), infralimbic and medial prefrontal cortex (anterior cingulate cortex) (Bregma 3.20 to 2.20 mm), and temporal cortex; hippocampal subregions CA1, CA2, CA3; and dentate gyrus (Bregma –3.60 to –4.30 mm), nucleus accumbens, as well as striatum (caudate and putamen) (Bregma 1.70 to –0.70 mm).
3.3.1 Receptor Autoradiography
Using quantitative in vitro receptor autoradiography, binding to NMDA receptors was performed with specific ligands such as glycine, spermidine, and MK-801 according to the method of Zilles et al. (64, 65). To remove endogenous ligands such as glutamate and glycine, preincubation of the glass-mounted cryostat sections was carried out with 50 mM Tris–HCl buffer (pH 7.5) for a total of 15 min (3×5 min) at 4°C. Binding assays were performed as triplicates in the same magnesium- and zinc-free buffer at 22°C for 60 min in the presence of 30 μM glycine and 50 μM spermidine for opening of receptor channels and 5 nM [3H]-MK-801 (dizocilpine maleate).
In two adjacent sections per region, non-specific binding was determined by adding 10–4 M (+) MK-801 to the incubation solution. Typically, non-specific binding of less than 5% of total binding was found under these conditions. Non-specific binding was subtracted from total binding to obtain specific binding. Tritium-labeled sections were exposed on [3H]-sensitive film (Hyperfilm®, Amersham, Germany) for 4 weeks. Autoradiographs were digitized using an image analysis system (Inter Active Systems, Interfocus, Germany) and a video camera (Nikon, Japan). The gray value images of the coexposed [3H] plastic standards (Amersham, Germany) were used to compute a calibration curve by non-linear, least-squares fitting, which defined the relationship between gray values and concentration of radioactivity. The plastic standards were calibrated to tissue standards prepared from homogenized brain tissue with known protein content in order to express binding site densities in fmol/mg protein. Concentrations of radioactivity were multiplied by (K D+c)/c to obtain B max values (K D = equilibration dissociation constants of the ligand binding kinetics, 5 nM for [3H]-MK-801; c = incubation concentration of labeled ligand).
3.3.2 In Situ Hybridizations
By in situ hybridization and quantitative real-time PCR, we measured mRNA transcripts (Fig. 4.3). To obtain the probes for in situ hybridization, RNA was prepared from postnatal day 2 rat cerebral cortex using a commercial kit (RNeasy, Qiagen, Germany), and cDNA was synthesized with Superscript II reverse transcriptase (Life Technologies, Germany) and oligo-dT primers (PerkinElmer, Germany). After PCR amplification (Promega, Germany) using specific primer sequences, the PCR product was cloned (Sure Clone Ligation Kit, Pharmacia, Germany) into the EcoRV site of pBluescript-SK. Sequence identity of the insert was confirmed by sequencing.
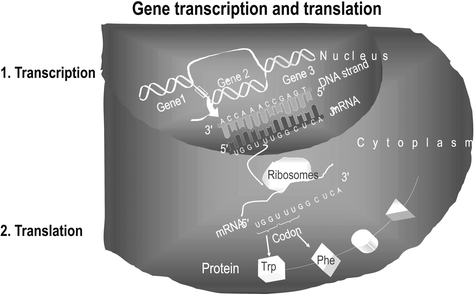
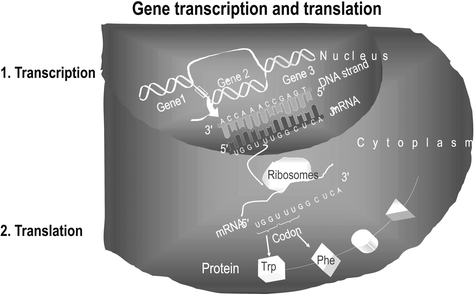
Fig. 4.3.
Base pairs are attached to the DNA strand and this messenger RNA represents the information of a gene. After transport to the cytoplasm it is translated into the protein.
Asymmetrically digested plasmids served as templates for in vitro transcription with T3 or T7 RNA polymerase (MBI Fermentas, Germany), respectively, to obtain antisense and sense cRNA probes. Efficacy of incorporation of radioactively labeled 35S-dUTP was measured and hybridizations of duplicate brain sections with antisense and sense probes in concentrations of 107 cpm/ml were performed for 16 h at 55°C in the presence of 50% formamide, 20 mM Tris, pH 7.5, 1x Denhardt’s solution, 1.25 mM EDTA pH 8.0, 100 mM DTT, 10% dextran sulfate, 2x SSC, 0.1% SDS, and 1 mg/ml yeast RNA hybridization buffer. After stepwise washings in 2x/1.5x/0.2x SSC dilutions, RNase A digest (10 μg/ml RNase A in 1.5x SSC), and dehydration, three slices (antisense) and one slice (sense) per region and animal were exposed to X-ray films (Biomax MR1) and coexposed with 14C plastic standards for 4–6 days to obtain radioactivity concentration in Bq/mg brain tissue. Autoradiographs were digitized using the image analysis system mentioned above (Fig. 4.4).
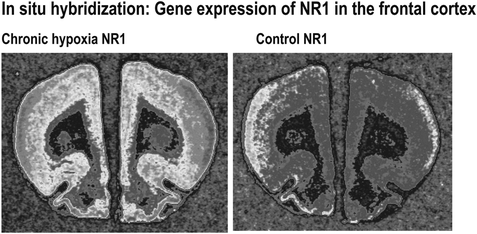
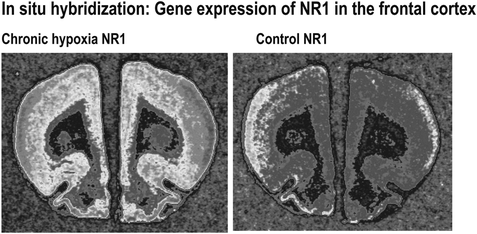
Fig. 4.4.
35S-stained rat brain sections and their analysis after exposure to sensitive films (in situ hybridization) allows the measurement of expression of a single gene (for example, NR1) in several delineated brain regions.
3.3.3 RNA Isolation and Reverse Transcription
One milliliter of Trizol was added to excised tissue and the tissue was homogenized by 30 strokes of trituration through a 27 gauge needle attached to a 1 ml syringe. After addition of 200 μl chloroform and thorough mixing, the solution was transferred to Phase-Lock tubes (Eppendorf) for phase separation. Aqueous supernatants were purified and concentrated by RNeasy mini columns (Qiagen). Quality control was performed by OD measurements in a NanoDrop ND-1000 spectrophotometer (260/280 nm >1.8) and by electrophoretic separation using an Agilent 2100 Bioanalyzer (R.I.N. values >7.0). Reverse transcription of purified total RNA was carried out using oligo-dT primers with T7 promoter sequence and Superscript II (Invitrogen).
< div class='tao-gold-member'>
Only gold members can continue reading. Log In or Register a > to continue
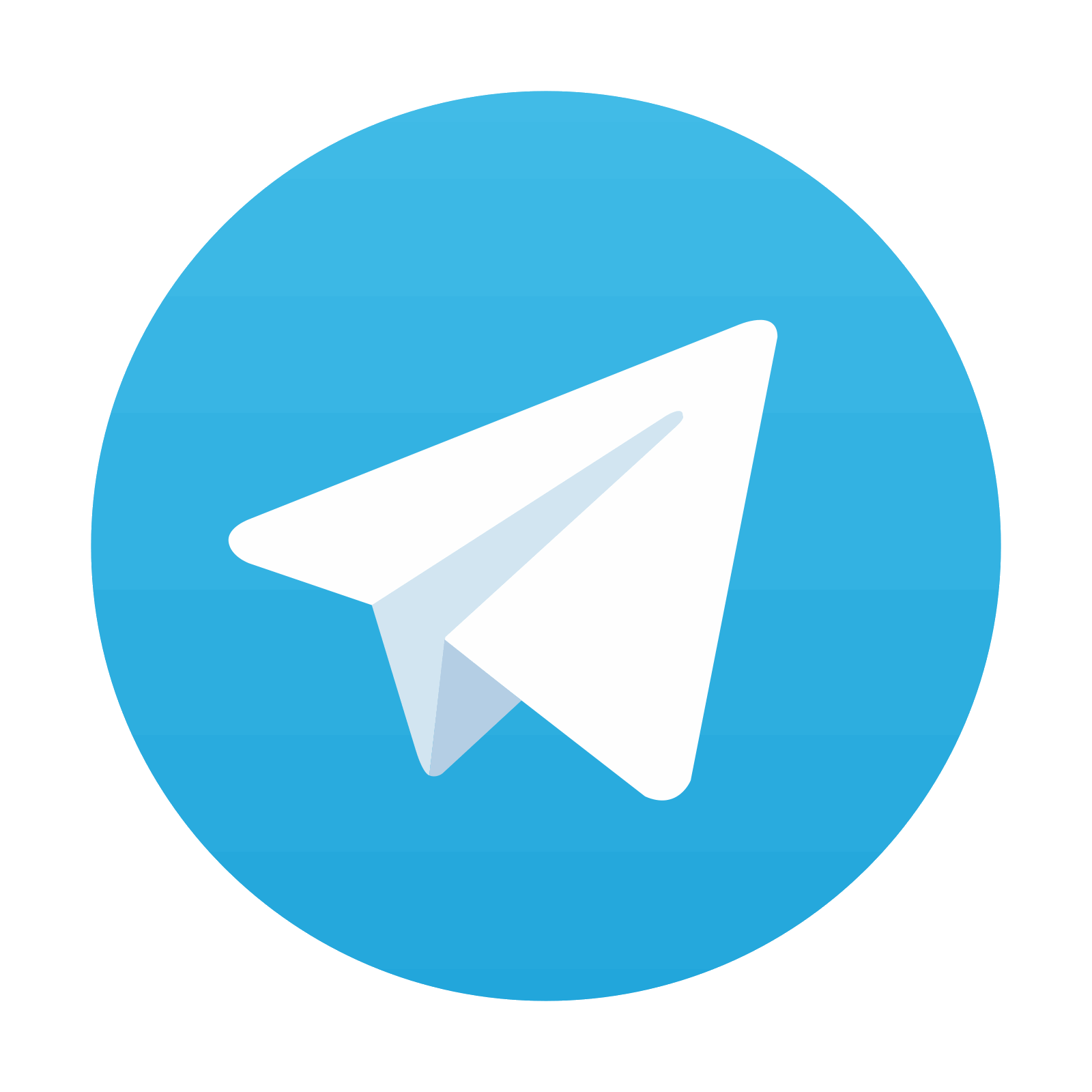
Stay updated, free articles. Join our Telegram channel
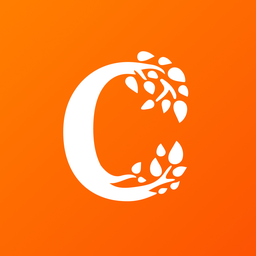
Full access? Get Clinical Tree
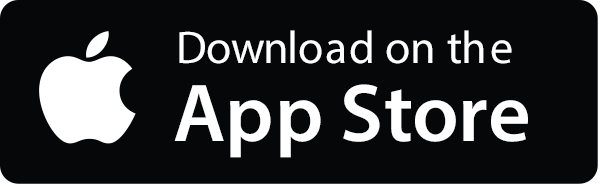
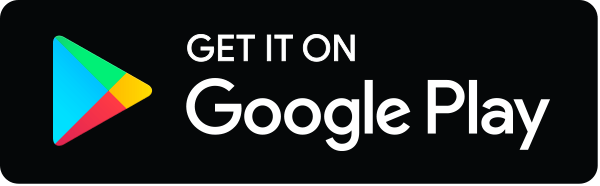