Fig. 1.
General methodology for the study of locomotion in cats with spinal lesions. With a complete lesion of the spinal cord at T13, cats are positioned with their forelimbs standing on a platform fixed above the belt, while the hindlimbs are free to walk on the moving treadmill. With a partial lesion, the cat is free to walk with all four limbs on the treadmill. Pairs of electromyographic (EMG) electrodes are implanted into multiple muscles (only one pair is represented here). The multipin EMG connector is cemented to the skull using dental acrylic. Reflective markers are placed on bony prominent landmarks of the left hindlimb to reconstruct angular excursions. The swing and stance phases of each cycle are determined from foot contacts and foot lifts.
The cat is first trained to walk on a treadmill at various speeds for several consecutive minutes. Once the animal can accomplish this task consistently, it is implanted with electrodes to record muscle activity (EMG, electromyography). All surgeries are done under general anesthesia with 1–3 % isoflurane. Electrodes made of stainless steel wire are soldered to and led subcutaneously from connectors that will later be cemented to the skull using dental acrylic (i.e., the headpiece). The insulation is removed from the end of the wires, which are sewn into muscle bellies for EMG. Bipolar cuff electrodes made of polymer (8) can also be placed around nerves to evoke reflexes using electrical stimulation. To deliver drugs intrathecally, a cannula can be inserted in the subarachnoid space with a capped opening fixed to the headpiece. Drugs that cross the blood–brain-barrier can also be delivered intraperitoneally (i.p.). During and for a few days after surgery, animals are given an analgesic, such as buprenorphine. The animals are monitored daily by trained personnel for signs of discomfort.
A few days after implantation, baseline data (e.g., EMG, kinematics) are obtained while the cat walks on the treadmill. Kinematic data are obtained by placing reflective markers on bony landmarks and captured with a video camera to reconstruct angular excursions during locomotion (see Fig. 1). Foot lifts and contacts of the four limbs are determined off-line from video recordings using custom-made software. Following a few weeks of baseline recordings, a spinal lesion is performed. The spinal lesion, which can be partial or complete, is made by making an incision above the desired segments and by removing some tissue (e.g., muscle, connective tissue) to expose 1–2 vertebrae. In the case of a spinalization or dorsal lesion, the dorsal part of the vertebral segment is removed (i.e., a laminectomy) to expose the spinal cord. The dura is carefully cut with small surgical scissors, and lidocaine is applied topically and injected within the spinal cord. The spinal cord is then cut using surgical scissors and an absorbable hemostat is packed within the gap to prevent regrowth. Muscle and skin are then closed in anatomical layers before anesthesia is discontinued. If the SCI is complete, the bladder must be expressed daily by the experimenters for the duration of the study. Some animals with incomplete SCI also require bladder expression for the first few days following the lesion. Depending on the experimental protocol, the cat can then be retrained to recover hindlimb walking (see Sect. 5.2 for more details on locomotor training).
The chronically implanted cat has contributed substantially to the field of SCI research, from fundamental and clinical perspectives. It was key in demonstrating that the spinal cord, devoid of supraspinal inputs, can generate a well-organized locomotor pattern with full weight bearing, plantar foot placements, and activation of hindlimb muscles. It has also shown that the chronically injured spinal cord can continue to adapt to different conditions, such as treadmill speed, various stimuli, and further injury. Some of the key findings and insights derived from the chronically implanted cat are discussed later on.
3.2 The Decerebrate Unparalyzed Cat
Acute terminal experiments are valuable because they permit procedures that are more invasive than what is possible in freely behaving animals. In acute experiments, the cat is decerebrated, meaning that tissue from the brain is removed, primarily from the cortex and rostral brain. During decerebration, the cortex is removed without damaging the thalamus, basal ganglia, and brainstem, so that cardiorespiratory functions are unaffected. It can be done by suctioning brain tissue or by removing the blood supply to specific regions (i.e., anemic decerebration). Following decerebration, anesthesia is discontinued and neuronal excitability within the remaining CNS returns, sometimes in an elevated state. Decerebration is performed to remove sentience so that invasive measures can be made in an excitable system that is not depressed by anesthesia. The type of decerebration can vary by transecting the neuraxis at different levels (e.g., decorticate, premammillary, and postmammillary), which have varying effects on the interactions between supraspinal and spinal structures. For example, a premammillary decerebration spares a region of the brainstem that is involved in generating spontaneous locomotor activity, whereas with a postmammillary decerebration electrical stimulation of the brainstem is required to elicit locomotion (9). The different types of decerebration in the context of locomotor control are reviewed in detail by Whelan (9).
In acute terminal experiments, several procedures can be performed, including electrical stimulation of circumscribed regions of the brainstem (9, 10), spinal cord (11–14), and other brain structures (15, 16). Peripheral nerves, dorsal roots, and ventral roots can also be stimulated to evoke or condition motor responses (17, 18). The EMG of several muscles can be evaluated at rest and during locomotion using percutaneous intramuscular electrodes. The limbs can also be immobilized and tendons can be attached to pullers and force transducers to stretch or vibrate specific muscles or to measure force generation (19, 20).
The decerebrate cat has been instrumental in our understanding of the descending control and sensory regulation of locomotion. Specifically, the decerebrate cat preparation established that walking was regulated by centers originating in the brainstem (reviewed by (9, 21, 22)). Complex interactions between cortical and subcortical structures shape the output from the brainstem to the spinal cord to initiate and regulate locomotion. Treadmill locomotion in the decerebrate cat was also used to demonstrate the importance of reflex pathways from group I ankle extensor afferents in regulating the duration of the stance phase and the stance-to-swing transition (23, 24).
3.3 The Decerebrate Paralyzed Cat
Some major breakthroughs in neuroscience were performed in the decerebrate paralyzed cat. The decerebration is the same as described in the previous section. Paralysis is achieved by injecting a drug intravenously, such as curare, which blocks transmission across the neuromuscular junction. As a result, no movement can occur and animals must be artificially ventilated to maintain respiratory function. Despite the absence of movement, locomotor-like activity can be recorded from muscle nerves, ventral roots, and/or by intracellular recordings from spinal interneurons or motoneurons. Recording locomotor-like activity in the absence of overt movement has been termed “fictive locomotion.” Although the absence of movement eliminates phasic sensory feedback, which is important for regulating locomotor output, the effect of specific inputs on the spinal locomotor network can be evaluated in relative isolation. Fictive locomotion was first recorded in the adult cat (25).
Fictive motor patterns can occur spontaneously or can be triggered by various types of stimulations (i.e., tactile, electrical, or pharmacological). During fictive locomotion, the extension and flexion phases correspond approximately to the stance and swing phases of walking. Figure 2 shows the EMG and electroneurography (ENG) of several hindlimb muscles and nerves, respectively, during treadmill walking (Fig. 2a) and during an episode of spontaneous fictive locomotion (Fig. 2b) in adult cats. During both types of locomotion, the burst in the knee flexor/hip extensor semitendinosus (St) muscle or the combined posterior biceps-semitendinosus (PBSt) nerve is of short duration and begins slightly earlier than the burst in other flexor muscles or nerves, such as tibialis anterior (TA, ankle flexor) and sartorius (Srt, hip flexor/knee extensor) during treadmill walking or TA and extensor digitorum longus (EDL, ankle flexor/toe extensor) during fictive locomotion. The similarity in the sequential activation of various nerves and muscles during fictive and real locomotion, respectively, indicates that a large part of the pattern is centrally generated, although supraspinal signals and sensory feedback certainly play a major role in shaping the final pattern during real movements through dynamic interactions with the spinal network (comprehensively reviewed in (26)).
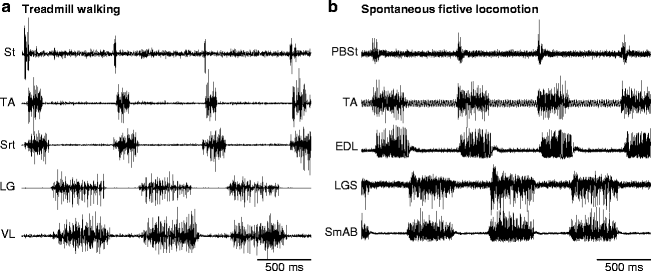
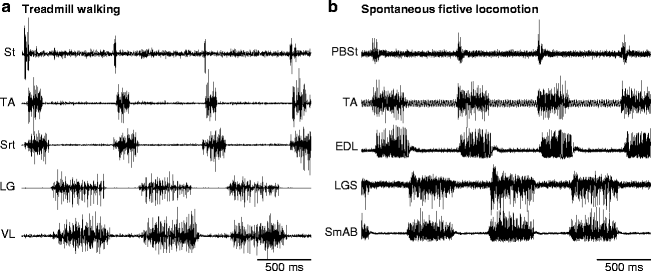
Fig. 2.
Pattern of activation during real and fictive locomotion in adult cats. (a) The electromyography of several muscles of the left hindlimb during treadmill walking at a speed of 0.4 m/s in an adult cat. (b) The electroneurography of several nerves of the left hindlimb during spontaneous fictive locomotion in an adult cat. St semitendinosus, TA tibialis anterior, Srt sartorius, LG lateral gastrocnemius, VL vastus lateralis, PBSt posterior biceps-semitendinosus, EDL extensor digitorum longus, LGS lateral gastrocnemius-soleus, SmAB semimembranosus-anterior biceps.
Another considerable advantage of the paralyzed preparation is that intracellular recordings can be made from spinal neurons, which requires a great deal of recording stability (i.e., very small to no movement). The first intracellular recording of a mammalian neuron, a spinal motoneuron, was made in the cat (27). Since that time, many different types of neurons within the spinal cord of the cat have been identified and recorded at rest, during fictive locomotion, and fictive scratch. At present, the number of identified neurons in the spinal cord of the cat constitutes the largest body of work of its kind in a mammalian species (for detailed reviews see (28, 29)). Evaluating the cellular properties of the spinal cord before and after SCI is important for targeted therapies.
4 Lesion Types and Recovery of Locomotion
The cat has been important in detailing the recovery of locomotion, as well as the deficits incurred following complete spinal lesions (i.e., spinalization) and various types of partial spinal lesions. Some of the studies that have investigated the recovery of locomotion after various types of spinal lesions are indicated in Table 1, which are grouped by lesion type and level of injury. Different descending pathways within the spinal cord are involved in different aspects of locomotion (for reviews see (21, 22, 26)). For instance, the reticulospinal pathway has a role in initiating and modulating the locomotor pattern, while the vestibulospinal pathway is more concerned with postural control. Cortico- and rubrospinal pathways are involved in volitional and anticipatory aspects of locomotion. Moreover, descending pathways originating in the brainstem release neurotransmitters, such as serotonin (5-HT) and noradrenaline (NA) that can have potent effects on spinal neuronal excitability. The location of these pathways within the spinal cord of the cat are well known (30, 31). The dorsolateral spinal cord contains cortico- and rubrospinal tracts and part of the medullary reticulospinal pathway. Part of the serotoninergic pathway from the Raphe nucleus is also located dorsolaterally. The dorsal columns, which convey sensory information to supraspinal structures are located in the dorsomedial spinal cord.
Table 1
Studies that have investigated the recovery of hindlimb locomotion following various types of spinal lesions. Studies are grouped according to the type of lesion and the level of transection. C, cervical; L, lumbar; T, thoracic
Type of lesion | Level of spinal lesion | Studies |
---|---|---|
Complete | T12–L1 | Robinson and Goldberger (1986a, b); Lovely et al. (1986); Carter and Smith (1986); Barbeau and Rossignol (1987, 1990, 1991); Barbeau et al. (1987, 1993); Lovely et al. (1990); de Guzman et al. (1991); Howland et al. (1995); Bélanger et al. (1996); Chau et al. (1998); de Leon et al. (1998, 1999); Bouyer et al. (2001); Giroux et al. (2003); Bouyer and Rossignol (2003); Frigon and Rossignol (2008a, 2008b, 2009) |
Partial—dorsal/dorsolateral | C2–C3 | English (1980) |
T6 | English (1985) | |
T10–T13 | English (1980, 1985); Zmyslowski et al. (1993); Gorska et al. (1993); Jiang and Drew (1996) | |
Partial—ventral/ventrolateral | T10–L1 | Gorska et al. (1990, 1993); Bem et al. (1995); Brustein and Rossignol (1998, 1999) |
Partial—lateral hemisection | C2 | Kato et al. (1983, 1984, 1985) |
T10–L3 | Murray and Goldberger (1974); Kato et al. (1983, 1984, 1985); Bregman and Goldberger (1983); Eidelberg et al. (1986); Kato (1989, 1990); Helgren and Goldberger (1993); Kuhtz-Buschbeck et al. (1996); Tester et al. (2008); Barriere et al. (2008, 2010); Frigon et al. (2009) | |
L4–S1 | Kuhtz-Buschbeck et al. (1996) | |
Dual—serial lateral hemisections | C2 then T6; T12 then T6 | Kato et al. (1983, 1984, 1985) |
Dual—lateral hemisection + myelotomy | L2–L3 then caudal myelotomy | Kato (1989, 1990) |
Dual—lateral hemisection + spinalization | T10–T11 then T12–T13 | Barriere et al. (2008, 2010); Frigon et al. (2009) |
The ventral spinal cord contains the pontine and medullary reticulospinal pathways and the lateral vestibulospinal tract. Part of the serotoninergic pathway from the Raphe nucleus and most of the noradrenergic pathway from the Locus Coeruleus are also located ventrally. Consequently, injury to specific regions or tracts of the spinal cord produces characteristic deficits, which have been well described in the adult cat for low thoracic injuries (i.e., T12–T13).
4.1 Complete Transection or Spinalization
A complete transection of the spinal cord, or spinalization, abolishes all descending inputs from structures rostral to the lesion (Fig. 3b). Complete SCI animal models have been instrumental in our understanding of the spinal control of locomotion. Numerous studies have investigated how the hindlimb locomotion recovers with time, training, and/or drug injections in kittens (32–37) and adult cats (38–56). With locomotor training, which is discussed later on, hindlimb walking (i.e., spinal locomotion) can recover after a minimum of 3–4 weeks. Figure 4 shows an example of kinematics and EMGs during treadmill locomotion at 0.4 m/s before (gray) and after (black) spinalization in the same cat (54). After spinalization, step length (Fig. 4a, b) and the duration of the locomotor cycle are generally reduced. In this cat, the duration of the cycle was 1,079 and 684 ms before and after spinalization, respectively. Thus, to maintain the same treadmill speed, the hindlimbs of the spinal cat must walk with a faster rhythm. Maximal angular excursions are generally reduced during spinal locomotion (Fig. 4c). The timing of most muscles is generally similar before and after spinalization, whereas the amplitude of the activity in flexor muscles typically increases while that of extensors decreases, although this varies across extensors and between animals (Fig. 4d). However, there are some notable changes in timing that are frequently observed. In particular, the delay in the onset of the knee flexor St activity with the hip flexor Srt is reduced, and both muscles can discharge simultaneously.
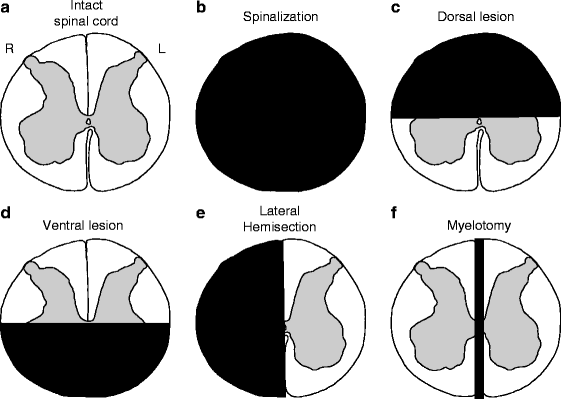
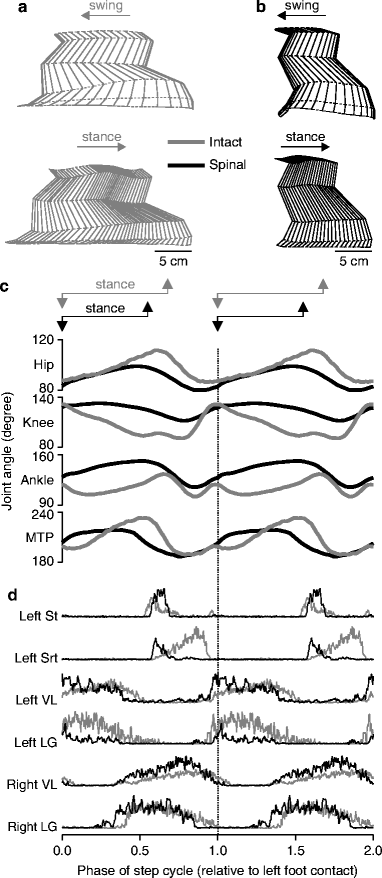
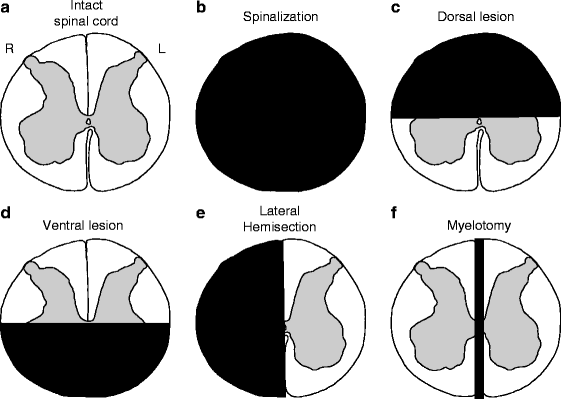
Fig. 3.
Schematic illustration of different spinal lesions performed in the cat. (a) A transverse view of the spinal cord at low thoracic levels. (b) A transection or spinalization of the spinal cord, which completely sections a small portion of the spinal cord in the transverse plane, effectively isolating all structures located caudal to the lesion. (c) A bilateral dorsal lesion, which removes the dorsal half of the spinal cord. (d) A bilateral ventral lesion, which removes the ventral half of the spinal cord. (e) A lateral hemisection, which removes one side of the spinal cord. (f) A myelotomy, which splits the spinal cord along the midline disconnecting the right and left sides.
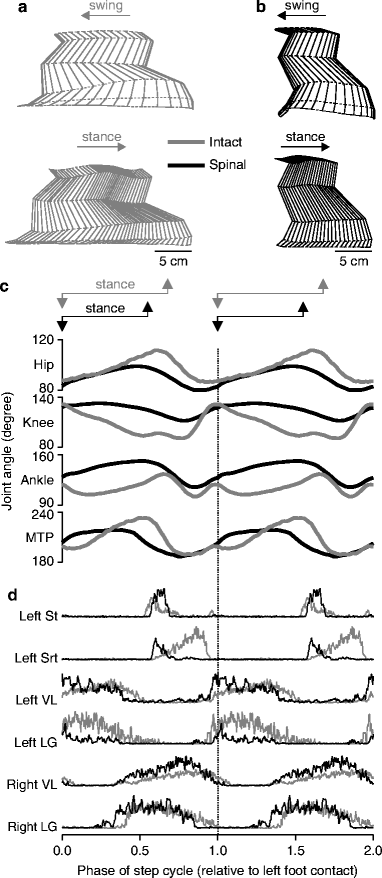
Fig. 4.
Kinematic and electromyographic data of a cat during treadmill locomotion at a speed of 0.4 m/s before and after spinalization. (a) Reconstruction of the left hindlimb as it moves during locomotion. Note that to prevent overlap of the stick diagrams, each one is displaced by an amount equal to the displacement of the foot along the horizontal axis. (b) Angular excursions of the hip, knee, ankle, and metatarsophalangeal (MTP) joints of the left hindimb before (gray) and after (black) spinalization in the same cat, normalized to Fig. 4. (continued) left foot contact. Stance is measured from left foot contact to left toe off. (c) Rectified, averaged, single burst EMG traces for selected muscles during intact (gray) and spinal (black) locomotion in a cat. Each trace is the average of 15–20 cycles, shown twice, normalized to left foot contact. Srt sartorius, St semitendinosus, LG lateral gastrocnemius, VL vastus lateralis.
4.2 Dorsal/Dorsolateral Lesions
Dorsal lesions of the spinal cord disrupt the dorsal columns and the cortico- and rubrospinal tracts, depending on the extent and location of the lesion (Fig. 3c). Dorsal lesions of the spinal cord have been used in numerous studies to evaluate the recovery of quadrupedal locomotion (57–61). In adult cats, voluntary quadrupedal locomotion recovers within 10 days after dorsal lesions (61). Bilateral damage to the dorsal/dorsolateral spinal cord at low thoracic levels results in longer steps, paw drag during the early swing phase, and an impaired ability to step over obstacles. Paw drag can persist in cats with more extensive lesions. With large lesions, the duration of the cycle and of the swing phase can increase. Posture and the coupling between hindlimbs are only transiently impaired following a dorsal lesion. The delay in the onset of the knee flexor St activity with the hip flexor Srt is reduced and both muscles can discharge simultaneously, similar to what is observed following spinalization.
4.3 Ventral/Ventrolateral Lesions
Ventral lesions of the spinal cord disrupt vestibulospinal and reticulospinal tracts, as well as serotoninergic and noradrenergic pathways. Ventral lesions have been studied in fewer studies (62–66) because they are harder to perform selectively. Following damage to the ventral/ventrolateral spinal cord at low thoracic levels, there is an initial period with loss of hindlimb walking and weight support that depends on the extent of the lesion (63–65). After a few days to a few weeks, hindlimb locomotion and weight support gradually recover. Despite the recovery of quadrupedal locomotion, lateral stability remains poor. Moreover, the coupling between the fore- and hindlimbs was altered following ventral lesions, as the frequency of the locomotor rhythm differed at both girdles in the most severely injured cats. In addition, the deficits were more pronounced during incline treadmill walking.
4.4 Lateral Hemisections
A lateral hemisection is made by sectioning half of the spinal cord on the left or right side at a specific segment, which is akin to a Brown-Séquard SCI (see Fig. 3e). The lateral hemisection is a popular model to investigate the recovery of hindlimb locomotion in cats (67–80). During the first few days after lateral hemisection, the hindlimb on the side of injury displays flaccid paresis and drags on a moving treadmill belt (70, 71, 75, 76, 80). If the lateral hemisection is performed at cervical levels, both the forelimb and hindlimb on the side of the lesion become paretic for approximately 1 week (70, 71). Animals require assistance for hindquarter support and body equilibrium during locomotion, although the deficits can vary between animals. Within 2 weeks, hindlimb weight bearing recovers and the affected hindlimb progresses from a passive crutch with minimal limb excursion to an active locomotor pattern (75, 76). With larger lesions, the coordination between the fore- and hindlimbs (i.e., intergirdle coordination) can be permanently lost, which is characterized by a different rhythm at the two girdles (80). The strategy used to adapt quadrupedal locomotion after lateral hemisection varies between cats and appears to depend on the presence or loss of intergirdle coordination.
4.5 Dual Spinal Lesion Paradigms
Three different types of dual lesion paradigms are illustrated in Fig. 5. A few studies in cats have used two spinal lesions separated in time (i.e., staggered) to determine what structures might be responsible for the recovery of hindlimb locomotion after SCI. For instance, Kato and colleagues (70, 71) performed a unilateral hemisection at C2 or T12, which was followed several weeks later by a second unilateral hemisection on the opposite side of the spinal cord at mid-thoracic levels (Fig. 5a). Such staggered lateral hemisections disrupt long supraspinal and propriospinal pathways. In all cats, a voluntary quadrupedal locomotion recovered within a few days to a few weeks. However, the coordination between the fore- and hindlimbs was permanently lost. Kato also performed a hemisection at L2–L3, which was followed a few weeks later by a caudal myelotomy (73, 74), which is a longitudinal split along the midline of the spinal cord (Figs. 3f and 5b). The hemisection followed by myelotomy isolates the spinal cord controlling one hindlimb. With such lesions, locomotion initially consists of a tripod gait involving the forelimbs and the nonisolated hindlimb. A few days to a few weeks later, the isolated hindlimb recovers its walking ability, although the coordination between hindlimbs is lost.
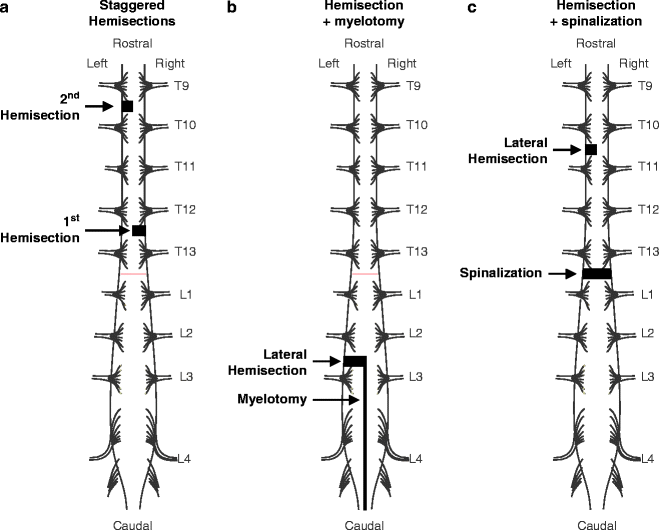
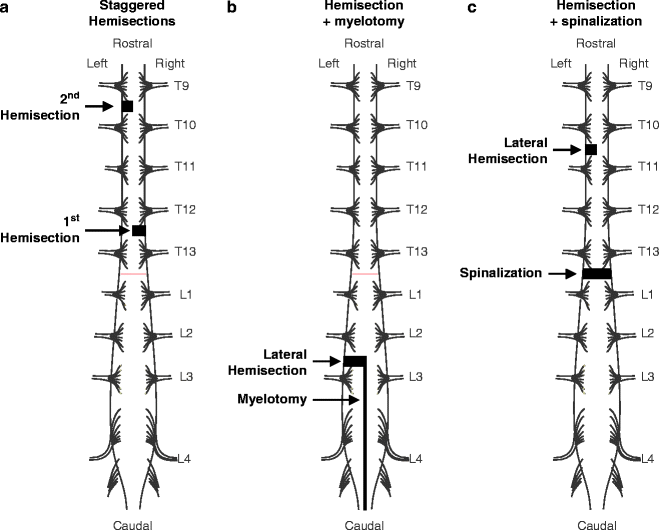
Fig. 5.
Dual spinal lesion paradigms in the cat. (a) Staggered hemisections. In the example shown in the figure, a lateral hemisection was made at T12 on the right side followed several weeks later by a mid-thoracic hemisection on the left side (70, 71). (b) A lateral hemisection followed by a caudal myelotomy. A hemisection was made at L2–L3 and was followed several weeks later by longitudinally splitting the spinal cord along the midline from the hemisection to the end of the cauda equina (73, 74). (c) A lateral hemisection at T9–T10 followed several weeks later by a complete transection 2–3 segments below at T12–T13 (78–80).
More recently, another type of dual spinal lesion paradigm was used (78–80), which consisted of a lateral hemisection at low thoracic levels followed several weeks later by a complete spinal transection (Fig. 5c). All cats recovered a voluntary quadrupedal locomotion following the hemisection. What was most remarkable was that following the spinalization, hindlimb locomotion spontaneously recovered within hours, a process that normally takes 3–4 weeks of intense treadmill training in chronic spinal cats. The underlying mechanisms involved in locomotor recovery with dual spinal lesion paradigms are discussed later on.
Therefore, different types of spinal lesions produce characteristic deficits that reflect in part damage to specific pathways. What is clear, however, is that no descending pathway is essential for the recovery of a voluntary quadrupedal locomotion following an incomplete SCI.
5 Key Findings
Many important findings for SCI research were first reported in the cat. The following sections summarize some of the seminal studies in the cat, highlighting the importance of their findings for SCI research.
5.1 The Spinal Origin of Locomotion: The Central Pattern Generator
Arguably, the most important discovery of the twentieth century for the control of walking was the demonstration that the spinal cord could generate the basic rhythm of locomotion by itself, which was first done in the adult cat. Thomas Graham Brown, a Scottish physiologist, transected the spinal cord of adult cats at the 12th thoracic (T12) segment and performed an extensive deafferentation of the hindlimbs by sectioning muscle nerves, thus abolishing supraspinal and peripheral inputs to the spinal cord (81). Only the nerves to the two test muscles, the gastrocnemius (ankle extensor) and tibialis anterior (TA, ankle flexor), were left intact, and sensory inputs from these muscles to the spinal cord were abolished by sectioning their dorsal roots. Despite the absence of inputs to the spinal cord, rhythmic alternating activity of the ankle extensor and flexor muscles occurred, which was evaluated by recording movement of the tendons of both muscles. This groundbreaking demonstration indicated that the generation of a rhythmic locomotor-like pattern is intrinsic to the spinal cord. The work by Brown is summarized in an excellent review article (82).
Despite what now seems like a clear demonstration for a spinal origin of locomotion, Brown’s findings did not garner much support among his peers. The prevailing view that locomotion was of “reflex” origin (83), persisted into the 1960s. It was not until the work by Anders Lundberg and his colleagues in Sweden on spinalized cats (84–87) that Brown’s findings resurfaced and became widely accepted. The assumption that an autonomous locomotor network also exists within the human spinal cord has greatly impacted therapeutic interventions in human SCI patients, although a definitive experimental demonstration of a spinal locomotor CPG in humans will never occur. For reviews discussing the existence of a spinal locomotor CPG in humans, see (88–90).
The spinal CPG for locomotion is also largely genetically determined, as kittens spinalized early after birth, before performing any walking movements, will express spinal locomotion (32–34). However, some elements of the spinal locomotor CPG are malleable and most likely contribute to the recovery of hindlimb walking after SCI (91). Experimental data from cats have been used to conceptualize or model the organization of the spinal locomotor CPG (92–96). At present, the functional organization of the mammalian locomotor CPG remains elusive, and extensive work in rodents and cats is ongoing. Therefore, a system of interconnected interneurons within the spinal cord organizes the basic pattern of locomotion, which can function autonomously from supraspinal structures following a complete SCI to restore walking ability. However, it should be emphasized that the CPG after complete SCI interacts with tonic and phasic sensory inputs from peripheral receptors located in joints, muscles, and skin. Thus, a better term is spinal “pattern generator (PG)” to denote an intrinsic spinal neuronal network that produces locomotor activity with inputs from the periphery. As discussed later on, the spinal locomotor PG is also critical in the recovery of locomotion following an incomplete SCI, even though supraspinal inputs can still access the spinal circuitry.
5.2 Locomotor Training
Locomotor training is based on the premise that providing sensory cues consistent with walking induces activity-dependent plasticity in the spinal network that generates locomotion (reviewed in (97, 98)). Locomotor training after a complete SCI was developed in adult cats during the 1980s concurrently in the laboratories of Serge Rossignol at the Université de Montréal (41) and of Victor “Reggie” Edgerton at the University of California at Los Angeles (38).
In the first few days after complete SCI, the legs of the cat are paretic, with little to no reflex movements or muscle spasms. During this period, the legs drag on the treadmill in an extended position with weak or absent spontaneous activity. Locomotor training consists of two experimenters moving the hindlimbs in a pattern consistent with walking and placing the plantar surface of the paws on the treadmill belt while it moves. The forelimbs are positioned on a stationary platform. After a few days, reflex movements and muscle spasms emerge and locomotor-like activity can be triggered by stimulating the perineal region (i.e., the skin located below the tail). At this point, the locomotor pattern is disorganized, with inappropriate contacts of the foot dorsum with the treadmill belt, short step lengths, inconsistent bouts of activity, and poor weight support. A Plexiglas separator is placed between the hindlimbs to prevent crossing of the legs. After a minimum of 3–4 weeks of locomotor training, 3–5 times a week, cats regain the ability to generate an organized hindlimb walking pattern with plantar foot contacts and weight support (38, 41, 42). However, postural control is permanently lost and the experimenter must hold the tail of the animal to provide equilibrium. The time course of recovery and the quality of spinal locomotion vary from one cat to another.
It was shown, in the adult cat, that functional recovery after complete SCI is specific to the type of training (99). For instance, cats that were trained to stand performed poorly at walking, whereas cats that were trained to walk had weak standing capacity. However, based on personal observations, a combination of stand and locomotor training works bests for locomotor recovery (unpublished observations). Combining stand and locomotor training appears to act synergistically, most likely by strengthening hindlimb extensor muscles, which is key to recovering weight support. The Edgerton group also showed that the spinal cord can “remember.” For example, when spinal cats were locomotor-trained for 12 weeks and training was then stopped for 12 weeks, the capacity for hindlimb walking diminished considerably (99). However, if locomotor training was restarted, hindlimb walking returned much more rapidly than initially observed. Therefore, locomotor training can facilitate the recovery of hindlimb walking following complete SCI, training appears to be task-specific, and the spinal cord devoid of signals from supraspinal structures can “remember.”
5.3 The Importance of Sensory Inputs for Locomotor Recovery
Although Sherrington’s original hypothesis that locomotion was generated by a reflex mechanism was inaccurate, it is evident that inputs from reflex pathways are critical in shaping the locomotor pattern through dynamic interactions with the spinal locomotor CPG (comprehensively reviewed in (26)). Moreover, sensory feedback to the spinal cord after SCI is critical for functional recovery, which was first made evident in the cat. For instance, early after spinalization, stimulating the perineal region facilitates the emergence of locomotion in adult cats. The skin from the perineum is thought to provide general excitability to the spinal locomotor network. If sensory inputs to the spinal cord are abolished before SCI, either by sectioning muscle nerves (56, 100), cutaneous nerves (53), or dorsal roots (101), the recovery of spinal locomotion is compromised (e.g., poor weight support, disorganized locomotor pattern). Some animals denervated before spinalization will not recover spinal locomotion (56). It thus appears that the integrity of sensory pathways at the time of SCI is critical for functional recovery thereafter.
Some sensory cues have more important roles during locomotion in the cat than others. For example, the position of the hip at the end of stance (102) and the amount of loading on ankle extensors (23) influence the stance-to-swing transition. Proprioceptive inputs from hip flexors or ankle extensors regulate phase transitions and can entrain the rhythm during fictive locomotion in adult cats (103–111) and/or treadmill walking in unparalyzed decerebrate cats (112–117). Experimental studies in humans successfully translated some of the cat data, primarily for sensory inputs from ankle extensors (118–120), thus showing the importance of sensory cues during bipedal human walking. The importance of hip position and ankle loading is stressed during rehabilitative walking in human spinal cord-injured patients (98).
Inputs from the skin, particularly from the foot, also have specific functions during locomotion. For example, mechanically stimulating the dorsum of the foot during the swing phase evokes a coordinated response that removes the stimulated limb from the obstacle in intact and spinal cats (121, 122), as well as humans (123–125). Removing skin inputs from the foot by sectioning the five cutaneous nerves produces only transient deficits during treadmill locomotion in intact cats (126). However, after complete SCI, these same cats were unable to correctly place their paws on the plantar surface despite several weeks of locomotor training (53). In addition, if the cutaneous denervation was performed after spinalization, some locomotor deficits appeared, such as paw drag during swing, but only once all skin inputs from the foot were removed. Therefore, inputs from the skin of the foot become increasingly important for locomotion after SCI.
< div class='tao-gold-member'>
Only gold members can continue reading. Log In or Register a > to continue
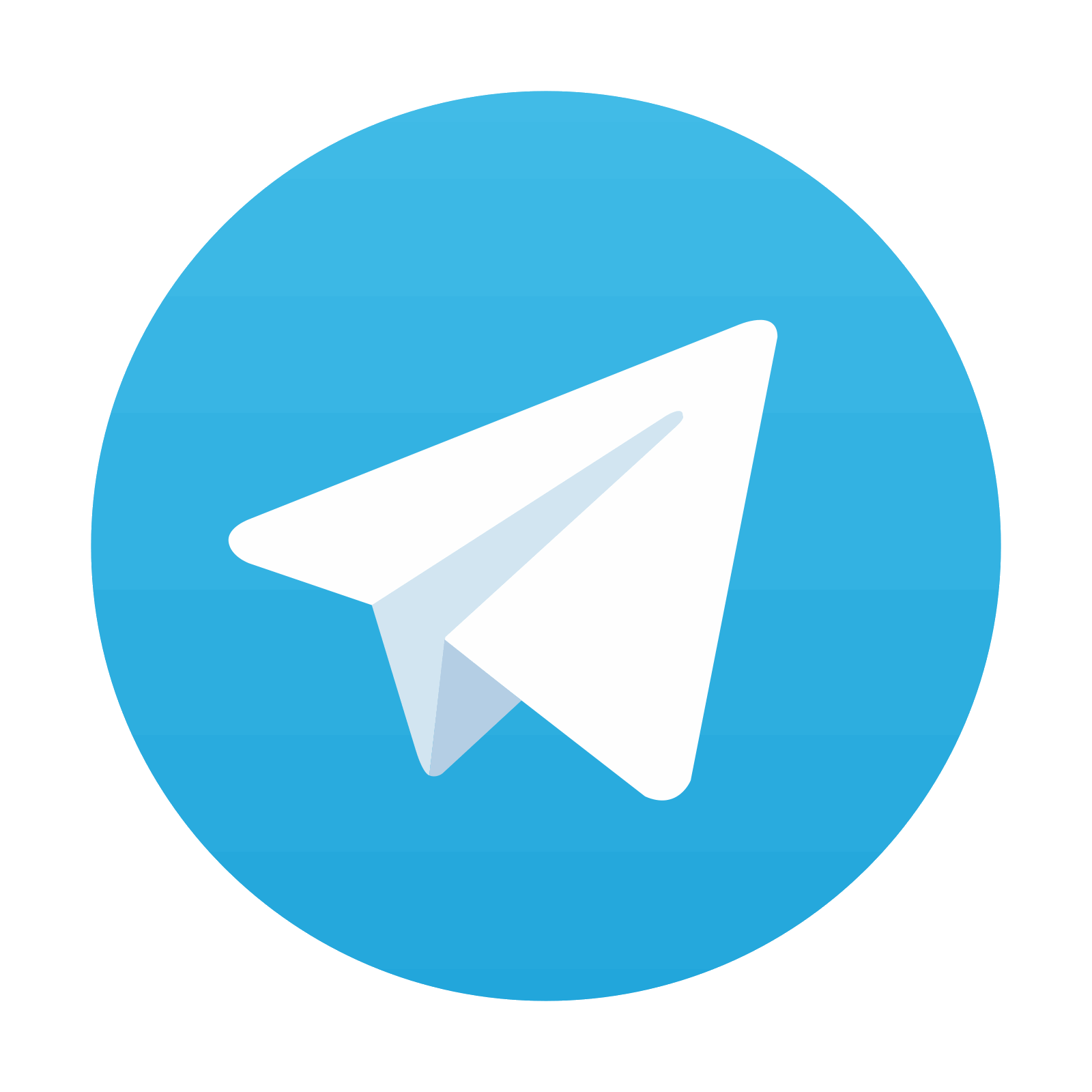
Stay updated, free articles. Join our Telegram channel
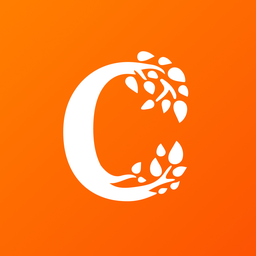
Full access? Get Clinical Tree
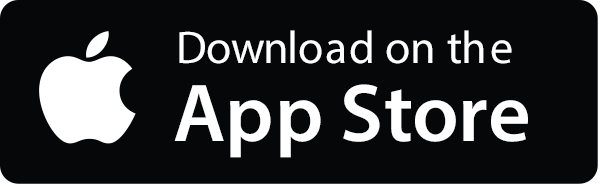
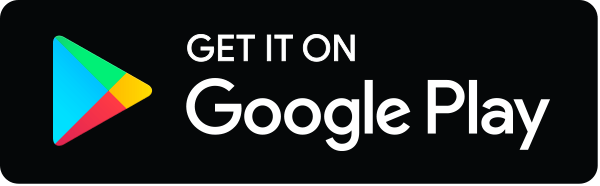