Lester Mandelker and Peter Vajdovich (eds.)Oxidative Stress in Applied Basic Research and Clinical PracticeStudies on Veterinary Medicine10.1007/978-1-61779-071-3_6
© Springer Science+Business Media, LLC 2011
Oxidative Stress in the Spinal Cord of Dogs and Cats
(1)
Small Animal Surgery, College of Veterinary Medicine, Oregon State University, Corvallis, OR 97331-4801, USA
Abstract
Oxidative stress in spinal cord disease is considered a secondary mechanism of injury following a primary traumatic event such as vertebral fracture or intervertebral disk protrusion (intervertebral disk disease). The primary spinal cord injury often results in decreased perfusion of the spinal cord due to compression from bone, disk, hematoma, or granuloma. This decreased blood flow to the cord, or ischemia, causes neutrophils, macrophages, and eosinophils to release reactive oxygen species among other inflammatory mediators. Oxidative enzymes are upregulated in the spinal cord following any injury and are a major source of oxidative damage for weeks by stimulating inflammatory cells to release more reactive oxygen species, perpetuating the cycle of oxidative damage. A multiarmed therapeutic approach may be needed in the treatment of spinal cord injury that encompasses all or at least several of the major mechanisms of injury, namely, glutamate-mediated excitotoxicity, increased intracellular Ca2+, and oxidative stress. These mechanisms lead to cell death through a loss of energy production, damage to nucleic acids and proteins, and apoptosis. Experimentally and in clinical trials, corticosteroids and N-acetyl-cysteine (NAC) have had limited success. Other free radical scavengers such as derivatives of acetylsalicylic acid and sulfasalazine, or aldehyde free radical scavengers are currently being investigated.
Keywords
CanineFelineDogCatSpinal cord traumaReactive oxygen speciesIsoprostanesIschemia reperfusionSecondary spinal cord injuryAcreolinHydralazineN-acetyl-cysteineOxidative stress is defined as an excess of reactive oxygen species (ROS) or a relative insufficiency of antioxidant defense mechanisms. ROS are generated from electron leakage from electron transport chains, inflammatory cells, UV light, drugs including chemotherapeutics, cigarette smoke, ozone and others. Oxidative stress in spinal cord disease is considered a secondary mechanism of injury following a primary traumatic event such as vertebral fracture or intervertebral disk protrusion (intervertebral disk disease, IVDD). The primary spinal cord injury often results in decreased perfusion of the spinal cord due to compression from bone, disk, hematoma or granuloma. This ischemia causes neuronal intracellular concentrations of ATP to decline, which then stimulates neurons to release the excitatory neurotransmitter glutamate [1]. Glutamate initiates a pathogenic cascade that results in cellular overload of calcium and increased production of ROS.
Neuronal ischemia also incites an inflammatory response with recruitment of neutrophils and macrophages and upregulation of cellular adhesion molecules. Neutrophils, macrophages and eosinophils release ROS among other inflammatory mediators. The free radical O2− is produced mainly by the enzyme nicotinamide adenine dinucleotide phosphate (NADPH) oxidase whose subunits are upregulated to form the active enzyme in the presence of proinflammatory cytokines [2, 3]. NADPH oxidase is upregulated in the spinal cord following injury and is thought to be a major source of oxidative damage for weeks following the primary injury [4]. ROS cause many secondary effects in the spinal cord following primary injury, including recruitment of additional inflammatory cells, which release more ROS, and the cycle of ROS-mediated neuronal damage is perpetuated [5].
Ischemia-reperfusion injury occurs as part of secondary injury in the spinal cord or with management of the injury such as surgical decompression. Reperfusion following ischemia in the spinal cord results in increased ROS production by mitochondria and stimulation of the enzyme xanthene oxidase to produce more ROS [1, 6, 7]. Peroxidation of lipids generates more reactive species including free radicals and reactive aldehydes such as malondialdehyde, 4-hydroxynonenal, and acrolein which bind to glutathione and result in additional oxidative stress [8–12].
The spinal cord is especially sensitive to oxidative stress because there are high levels of polyunsaturated fatty acids (PUFAs) present in the membranes of neurons and oligodendrocytes such as linoleic acid and arachidonic acid. The PUFAs react with ROS in cellular membranes resulting in propagation of new radicals. Lipid peroxidation will continue until all PUFAs are depleted or the free radicals interact with an antioxidant within the membrane, such as vitamin E [13]. Because lipid peroxidation is self-perpetuating, severe damage to neuron membranes results in alterations in ion channels, including calcium with an increase in intracellular calcium, eventually leading to apoptosis [14].
F2 isoprostanes (15F2t isoprostane or 8-isoprostaglandin F2α) are a class of free radical catalyzed products of the arachidonic acid pathway produced independently of cyclo-oxygenase enzymes in vivo. They have a high sensitivity and specificity for assessing oxidative stress in disease states and can be measured in the urine to assess lipid peroxidation [15]. In dogs with spinal cord injury from acute disk herniation, F2 isoprostanes significantly increase, indicating lipid peroxidation and oxidative stress occur in dogs with spinal cord injury [16].
Following the primary injury, secondary injury is also mediated by the interaction of ROS with nitric oxide (NO). NO production increases following spinal cord injury due to a rise in nitric oxide synthase enzymes iNOS and eNOS [4]. NO generation continues to be elevated for at least 14 days following spinal cord injury [4, 17]. NO and ROS react together to form highly reactive and cytotoxic molecules including peroxynitrite (ONOO−) and peroxynitrous acid (ONOOH) which damage lipids, proteins and nucleic acids [18]. Nitrotyrosine, a marker of superoxide–NO interaction remains elevated for at least 14 days following spinal cord injury, indicating that nitrosative and oxidative stress continue for at least weeks following the primary injury [4, 17].
Reperfusion injury following an ischemic event is particularly damaging to the spinal cord because the spinal cord is subject to severe lipid peroxidation with high levels of PUFAs and because the neurons contain high numbers of mitochondria, which are susceptible to ROS and produce ROS following ischemia. Up to 33% of humans develop paraplegia following thoracoabdominal aorta surgery due to ischemia and reperfusion injury following temporary aortic cross-clamping [19]. Indeed, in some patients, there is delayed onset of paraplegia one to five days following aortic cross-clamping and this neurological dysfunction is thought to be due, in large part, to ROS-mediated damage and lipid peroxidation [20, 21]. In dogs following acute spinal cord injury from intervertebral disk herniation, secondary mechanisms of injury including lipid peroxidation begin within four h of the primary injury from the disk and peak four days later [22]. During this period, demyelination, axonal degeneration and myelomalacia occur in regions inside and outside the primary zone of trauma [23–25]. ROS-induced damage to neurons is not limited to lipid peroxidation-mediated inflammation and cell death. ROS also damage proteins and DNA resulting in cellular dysfunction and potentially carcinogenesis [26].
Because oxidative stress can decrease myelin production and cause axonal degeneration, its role in degenerative myelopathy has been investigated in dogs [27, 28]. Degenerative myelopathy or canine degenerative radiculomyelopathy is a progressive degenerative disease of the thoracolumbar spinal cord of dogs for which no treatment exists and is believed to be hereditary in nature [29, 30]. Production of the metabolite of lipid peroxidation, 8-isoprostane, in the cerebral spinal fluid of Pembroke Welsh corgis with degenerative myelopathy was not different from control dogs [29]. In a study investigating German shepherd dogs with degenerative myelopathy, no defect in alpha-tocopherol transfer protein gene or expression was found, indicating that vitamin E metabolism in these dogs is unlikely to be a part of the pathogenesis of this disease [31]. In addition, vitamin E levels are not altered nor does supplementation with vitamin E have any effect on dogs with degenerative myelopathy [32]. At this time there is no evidence for a role of oxidative stress in degenerative radiculomyelopathy of dogs.
Endogenous antioxidant defense mechanisms such as antioxidant proteins, small molecule antioxidants and antioxidant enzymes react with ROS to donate a single electron to the free radical resulting in a more stable compound [33]. The spinal cord contains relatively low amounts of the antioxidant glutathione [24, 34]. Following trauma, the spinal cord is quickly depleted of its antioxidants including glutathione, vitamin E, and ascorbic acid [7, 24, 34]. The free radical scavenging enzyme superoxide dismutase increased within 24 h following the primary spinal cord injury and remained elevated for at least 14 days to counteract the increased production of ROS. Unlike superoxide dismutase, catalase and glutathione peroxidase remain unchanged in the first 24 h following injury, however, by 14 days they too have responded and are present in increased amounts compared to normal spinal cord [4]. The delayed response of some of the antioxidant enzymes coupled with the rise in free radical production may account for some of the continued secondary injury and deteriorating neurological function seen during the four days following the primary injury. In addition, continued oxidant stress may play a role in the final functional outcome of many dogs following spinal cord injury.
An important target of therapy for spinal cord injury is oxidative stress, however, whereas in vivo studies in animals have shown successful treatment with antioxidants, studies in human and dog clinical trials have failed to produce similar results [21, 35–37]. Methylprednisolone has been used widely for its antioxidant properties in the treatment of spinal cord injury. Improved outcome has been demonstrated in some studies, however, serious side effects can occur in dogs including gastrointestinal bleeding, wound infections and pneumonia [38–43]. In blinded placebo-controlled clinical studies in humans with spinal cord injury, methylprednisolone sodium succinate can improve motor outcome if administered within eight h of the trauma [38, 44, 45]. In a recent retrospective study on the use of glucocorticoids for thoracolumbar intervertebral disk herniation, dogs treated with no glucocorticoids, methylprednisolone, prenisolone or dexamethazone had no difference in short-term outcome based on their neurological scores [46]. In addition, dogs treated with dexamethazone were over three times as likely to have a clinical complication compared with dogs given no glucocorticoids or those treated with methylprednisolone or prednisolone [46]. Complications included urinary tract infections and diarrhea [46].
In the CNS, where levels of other endogenous antioxidants (i.e., catalase) are low, the cells rely more heavily on glutathione for protection and N-acetyl-cysteine (NAC) is its precursor [24, 47]. NAC also directly scavenges ROS and numerous laboratory and clinical studies have demonstrated a beneficial effect from NAC administration [48–53]. In a blinded randomized, placebo-controlled clinical trial NAC was administered intravenously immediately prior to decompressive hemilaminectomy in dogs with acute thoracolumbar disk herniation. NAC was unable to ameliorate the neurological deterioration that occurred from the spinal cord injury in dogs with intervertebral disk disease [37]. A recent study of spinal cord ischemia in rabbits identified that, although NAC treatment improved neurological status, neuronal cell damage was still significant [54]. In addition, in closed brain trauma although neuron morphology is somewhat protected [55], brain perfusion and edema are unaffected by NAC treatment [56]. In the dogs with acute thoracolumbar disk herniation, spinal cord edema may have been severe enough that outcome was not affected by NAC administration. Although NAC did not alter urinary 15F2t isoprostane excretion in dogs with IVDD-mediated spinal cord injury, it is possible that another antioxidant may have been more effective. The prostaglandin I2 analogue, iloprost, when combined with NAC, improves neurological outcome in rabbits subjected to spinal cord ischemia more effectively than NAC alone [54].
A multiarmed therapeutic approach may be needed in the treatment of spinal cord injury that encompasses all or at least several of the major mechanisms of injury, namely, glutamate-mediated excitotoxicity, increased intracellular Ca2+, and oxidative stress. These mechanisms lead to cell death through a loss of energy production, damage to nucleic acids and proteins, and apoptosis [57–65]. Neu200, a derivative of acetylsalicylic acid and sulfasalazine, inhibits NMDA receptor-mediated excitotoxicity and scavenges free radicals. Experimentally in rats, this compound improves functional recovery following acute spinal cord injury [57]. Another promising avenue of investigation for the treatment of spinal cord injury is through inhibition of free radical-generated aldehydes such as acreolin [8]. The acrolein scavenger hydralazine ameliorates secondary injury in the spinal cord, at least experimentally [66, 67].
Further understanding of the specific mechanism of oxidative stress in spinal cord injury and investigation into the best method for scavenging ROS may provide a better outcome in the future. Much work still needs to be done to understand how the various mechanisms of injury including oxidative and nitrosidative stress interact to produce the devastating effects of secondary injury in spinal cord trauma.
References
1.
Juurlink BH, Paterson PG: Review of oxidative stress in brain and spinal cord injury: suggestions for pharmacological and nutritional management strategies. J Spinal Cord Med 21:309–334, 1998.PubMed
< div class='tao-gold-member'>
Only gold members can continue reading. Log In or Register a > to continue
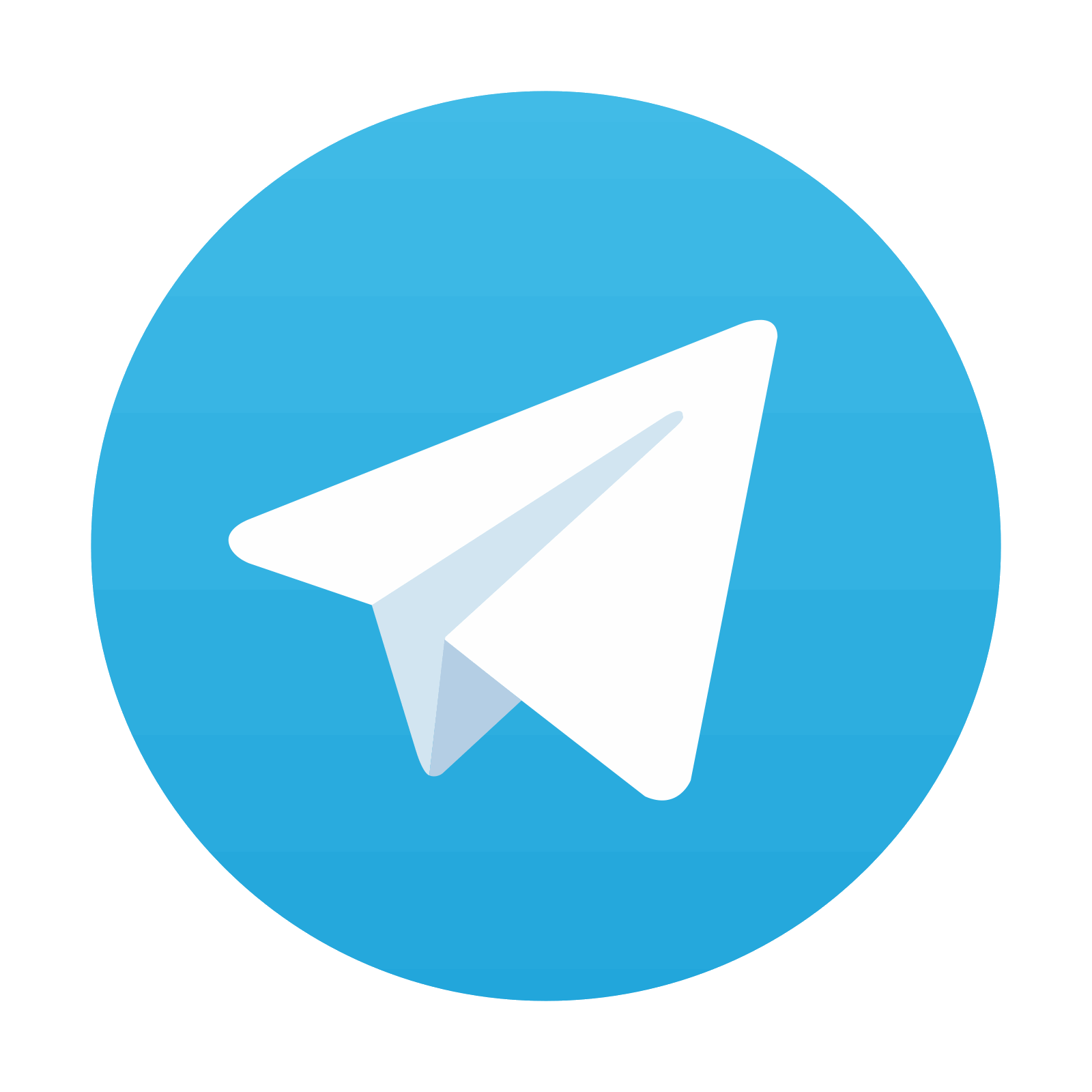
Stay updated, free articles. Join our Telegram channel
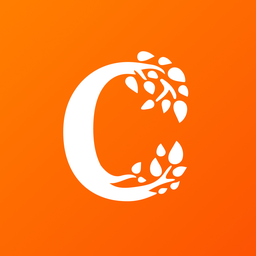
Full access? Get Clinical Tree
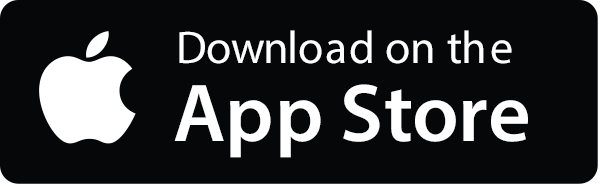
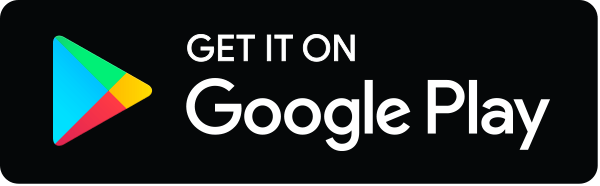