Fig. 1
Western immunoblot analysis of proteins from left ventricular and left atrial tissues. Representative Western immunoblots (a, b) showing specific peptide levels in homogenates of left atrium (LA) and left ventricle (LV) from control (C) and paced (P) dog hearts; (c) actin. Reproduced with permission from: [41]
Oxidative Stress and Cardiac Function
Oxygen serves as the critical terminal electron acceptor in the mitochondrial ETC; in its absence the ETC shuts down and cardiac demands for ATP are not met. Molecular oxygen is also central in both the formation of NO, a primary determinant of both vascular tone and cardiac contractility, and in the generation of ROS (and subsequent oxidative stress) as a significant by-product of energy metabolism during its sequential acceptance of electrons in the mitochondrial ETC. The end results of NO/redox disequilibrium have implications for cardiac and vascular homeostasis and may result in the development of atherosclerosis, myocardial tissue remodeling, and hypertrophy. ROS/RNS generation is also attributed to the transit from hypertrophic to apoptotic phenotypes, a possible mechanism of myocardial failure [42].
Endothelial dysfunction is a critical component in the systemic vasoconstriction and reduced peripheral perfusion that characterizes patients with HF [43]. Endo-thelial regulation of vascular tone is mediated mainly by NO. Increased oxidative stress in patients with HF may be related in part to decreased bioavailability of NO secondary to reduced expression of endothelial NO synthase (eNOS) and increased generation of ROS. These react with NO in the setting of decreased antio-xidant defenses that would normally clear these radicals, culminating in attenuated endothelium-dependent vasodilation particularly in patients with advanced HF. Moreover, abnormal production and/or distribution of ROS and reactive nitrosative species in blood creates oxidative and/or nitrosative stresses in the failing myocardium and endothelium.
As previously noted, the uncoupling of constitutive NOS leads to increased generation of superoxide (O2·−) and peroxynitrite (ONOO−). Peroxynitrite affects cardiovascular function and contributes to the pathogenesis of cardiac and endothelial dysfunction secondary to MI, chronic HF, diabetes, atherosclerosis, hypertension, aging, and various forms of shock. Moreover, pharmacological inhibition of xanthine oxidase (XO)-derived superoxide formation, as well as neutralization of peroxynitrite, or inhibition of PARP, may provide benefit in various forms of cardiovascular injury [23]. Increased oxidative stress resulting from an overproduction of superoxide mediates the dysregulation of S-nitrosylation of proteins at specific cysteine residues by RNS, a more selective modification of proteins than found with protein oxidation. This redox mechanism has been found to lead to altered myocardial excitation-contractility and vascular reactivity [23, 24].
In the vasculature, disease states such as hypertension and the activation of the renin–angiotensin–aldosterone system (RAAS) can result in reduced vascular NO, as NO synthase becomes uncoupled with the oxidative depletion of its cofactor tetrahydrobipterin (BH4), leading to the production of superoxide (O2·−) in lieu of NO [44]. Both NO and NOS have been implicated in the modulation of cardiac relaxation [45]. Using a series of carefully controlled invasive hemodynamic studies and noninvasive imaging methods, Silberman and colleagues recently studied a mouse model that developed diastolic dysfunction in the absence of systolic dysfunction or cardiac hypertrophy [46]. Cardiac tissues from these mice showed increased oxidation of BH4 as well as increased superoxide formation, reduced NO production, and dephosphorylated phospholamban. Feeding hypertensive mice BH4 improved cardiac BH4 stores, phosphorylated phospholamban levels, and the diastolic dysfunction. The investigators concluded cardiac oxidation, independently of vascular changes, could lead to uncoupled cardiac NOS and diastolic dysfunction. The heart preparations analyzed in this study contained both the eNOS and nNOS isoforms that are expressed within cardiac myocytes, in addition to the eNOS that is robustly expressed in cardiac endothelial cells, which may raise a challenge to separating the roles of cell- and isoform-specific NOS pathways at the molecular level. Nevertheless, the observation that BH4 and related redox pathways play a key role in cardiac diastolic function, represents an important addition to the growing appreciation of the salutary biological effects of BH4 in overall cardiovascular homeostasis [47, 48].
Taken together, studies that have explored the pathways whereby BH4 ameliorates diastolic dysfunction [46] or endothelial dysfunction [49] have provided indirect evidence that implicates the RAAS in the pathophysiological response. Angiotensin II modulates ROS production in diverse biologic tissues and has been shown to stimulate eNOS-derived O2− production, which in turn is suppressed by BH4 [49]. The vascular dysfunction seen in models of diabetes, hypercholesterolemia, and hypertension is associated with enhanced oxidation of BH4 to BH2 and can be restored in vivo by BH4 supplementation [47, 48, 50]. Moens et al. [51] have reported that administration of BH4 can attenuate the cardiac hypertrophy and myocardial fibrosis seen in a model of pressure overload, in association with an improvement in oxidative stress. These investigators provided evidence indicating that the effects of BH4 were due to effects on NOS pathways in cardiac myocytes but did not involve activation of cGMP-dependent pathways in the heart.
To further assess the role of renin-angiotensin-aldosterone system in mediating oxidative stress in HF, we recently studied the impact of angiotensin II type 1 receptor (AT1R) blockade on hemodynamics, LV remodeling, oxidative stress, and tissue expression of AT(1)R and angiotensin II type 2 receptors (AT2R) in a canine model of pacing-induced HF [52]. Animals were randomized to rapid right ventricular-pacing for 3 weeks to severe heart failure and treated with candesartan or placebo from day 3 onwards, or no pacing. Candesartan significantly reduced pulmonary arterial and LV diastolic pressure, LV end-diastolic and end-systolic volume and ascites, increased cardiac output, dP/dt, and ejection fraction, while reversing the marked increase in aldehydes, a marker of oxidative stress, observed in the placebo group (Table 1). Although candesartan did not alter LV AT1R protein expression compared to placebo or sham, it reversed the decrease in AT2R protein observed in the placebo group. We therefore conclude that in the pacing model of heart failure, chronic AT1R blockade attenuates hemodynamic deterioration and limits LV remodeling and dysfunction, in part by reversing oxidative stress and AT2R downregulation.
Table 1
Effect of angiotensin receptor blockade on LV tissue aldehyde concentrations (Reproduced with permission from Moe et al. [52])
Aldehydes(ρmol/100 mg) | Sham | Paced/placebo | Paced/candesartan |
---|---|---|---|
Saturated | |||
Heptanal | 148 ± 26 | 297 ± 54* | 172 ± 43*** |
Octanal | 177 ± 21 | 177 ± 32 | 197 ± 28 |
Decanal | 72 ± 3 | 77 ± 8 | 61 ± 9 |
Unsaturated | |||
t-2-Heptanal | 3,447 ± 420 | 3,557 ± 810 | 2,387 ± 485*** |
t-2-Octanal | 149 ± 18 | 205 ± 19* | 154 ± 19*** |
t-2-Nonenal | 210 ± 18 | 275 ± 36* | 168 ± 14*** |
t,t-2,4,-Nonadienal | 17 ± 4 | 24 ± 3 | 18 ± 4 |
4-OH-Hexanal | 1,469 ± 102 | 2,337 ± 168** | 1,728 ± 268*** |
4-OH-Nonenal | 693 ± 53 | 1,200 ± 175* | 1,189 ± 175 |
4-OH-Decanal | 5.7 ± 0.7 | 6.9 ± 0.2 | 5.8 ± 0.7 |
Malondialdehyde | 2,009 ± 108 | 14,125 ± 1,002** | 5,563 ± 874*** |
Antioxidant Therapy
ROS and cellular redox states regulate an extensive number of vital pathways in the myocardium, including energy metabolism, survival and stress responses, apoptosis, inflammatory response, and oxygen sensing. Gathered observations have shown that the role ROS play in ischemia and reperfusion injury, as well as the role that antioxidant therapy may play, varies whether one use whole organ and animal models or isolated cell models; findings are often contradictory, thereby explaining in part why clinical trials of antioxidants frequently have shown mixed results. The powerful cell-damaging ROS oxidants can be neutralized by an array of protective antioxidant scavenger enzymes, as well as by various lipid and water-soluble compounds including ascorbic acid, glutathione, thioredoxin, and α-tocopherol. The antioxidant enzymes are located in a variety of cellular compartments including the mitochondria (e.g., MnSOD, glutathione peroxidase, thioredoxin reductase, peroxisomes, e.g., catalase, microsomes, e.g., cytochrome p450) and in the cytosol (e.g., CuSOD and cytosolic thioredoxin reductase). In general, there are significantly lower levels of antioxidants in myocardial mitochondria than in liver mitochondria, but the consensus opinion is that the antioxidant capacity of the heart is generally sufficient to handle normal levels of ROS production, but not the greater ROS accumulation that occurs in myocardial ischemia [53].
A mitochondrial isoform of catalase with low specific activity has been found in rats [54, 55]. This mitochondrial catalase activity was detected in the heart but not in the liver or skeletal muscle and it appeared to increase during caloric-restricted diets and in the diabetic heart [56, 57]. The role that this enzyme plays has not been fully determined although there is evidence of its participation in the prevention of excess lipid peroxidation in myocardial ischemia [58]. On the other hand, a mitochondria-specific catalase has not been found in the heart of transgenic mice even after overexpression of the catalase gene [59]. SODs catalyze the removal of superoxide radicals by the formation of H2O2, however, GPx catalyzes the breakdown of H2O2 to water and oxidized glutathione (GSSG) by using reduced glutathione (GSH). Because GPx is located in both the mitochondria and cytosol, H2O2 can be removed from either compartment depending on the availability of glutathione. A small fraction of the total cellular pool of GSH is sequestered in the mitochondria by the action of a carrier that transports GSH from cytosol to the mitochondrial matrix [60]. Upon exposure to increased exogenous ROS, isolated perfused rat hearts are rapidly depleted of their antioxidant reserves, including those of SOD and GSH, rendering them more vulnerable to the action of oxidative injury [61].
Another important mechanism in the antioxidant reactions is the sequestering of iron and copper ions to keep them from reacting with superoxide or H2O2. The antioxidant dexrazoxane prevents site-specific iron-based oxygen radical damage by chelating free and loosely bound iron. In addition, it has been used as a cardioprotective drug against doxorubicin-induced oxidative damage to myocardial mitochondria in both humans and animals [62]. The antioxidant metal-binding protein metallothionein (MT) also provides cardioprotection by reacting with ROS produced by ischemia/reperfusion and doxorubicin treatment as found in studies with a cardiac-specific MT-overexpressing transgenic mouse model [63]. MT expression is also inducible within the heart (and other tissues) by TNF-α, IL-6, doxorubicin, and metals such as cadmium and Zn [63, 64], although its cardioprotective role has yet to be determined.
The uncoupling of mitochondrial respiration from OXPHOS ATP production, by either artificial uncouplers such as 2,4-dinitrophenol (DNP) or natural uncouplers such as laurate, fatty acids, and mitochondrial uncoupling (UCP) proteins, strongly inhibits O2·− and H2O2 formation in mitochondria [65, 66]. ROS production is favored when the mitochondrial membrane potential is above a specific threshold. Under conditions where the mitochondrial membrane potential is at its peak, for example, state 4 respiration, ROS production is increased. It is noteworthy that increased mitochondrial membrane potential slows electron transport through the respiratory chain, resulting in increased half-life of the ubiquinone free radical and the likelihood that electrons will interact with oxygen to form ROS [67]. Uncouplers prevent the transmembrane electrochemical H+ potential difference (∆ŭH) from being above a threshold critical for ROS formation by respiratory complexes I and III. This has been corroborated in transgenic mice in which UCP3 protein is lacking, resulting in enhanced ROS production and increased OS in heart and skeletal muscle [68], in transgenic mice with UCP1 overexpression [69], and in cardiomyocytes with UCP2 overexpression, in which ROS is markedly attenuated [70].
Pharmacological inhibition of xanthine oxidase-derived superoxide formation and neutralization of peroxynitrite or inhibition of poly(ADP-ribose) polymerase (PARP) have been reported to provide significant benefit in various forms of cardiovascular injury [71]. Using rat and mouse models of HF, beneficial effects of a novel ultrapotent PARP inhibitor have also been demonstrated by Pacher et al. [72]. The effect of INO-1001 on the development of HF induced by permanent ligation of the left anterior descending coronary artery was assessed, as was HF induced by doxorubicin, and acute HF induced by bacterial endotoxin. In the coronary ligation model, significantly depressed LV performance and impaired vascular relaxation of aortic rings were found; PARP inhibition significantly improved both cardiac function and vascular relaxation. In the doxorubicin model, a single injection of doxorubicin induced high mortality and a significant decrease in LV systolic pressure, +dP/dt, −dP/dt, stroke volume, stroke work, ejection fraction, and cardiac output. Treatment with the PARP inhibitor reduced doxorubicin-induced mortality and markedly improved cardiac function; on the other hand, PARP inhibition did not interfere with doxorubicin’s antitumor effect. In the endotoxin model of cardiac dysfunction, PARP inhibition attenuated the reduction in myocardial contractility elicited by endotoxin. Taken together, these data support the view that PARP inhibition may be an effective approach in the experimental treatment of various forms of acute and chronic HF.
Mitochondrial ROS and OS are implicated in the pathogenesis of the cardiac damage elicited by ischemia/reperfusion and the cardiomyopathies associated with Fridreich’s ataxia (FRDA) and doxorubicin [73, 74]. Several oxygen radical scavengers including CoQ10, vitamin E, dexrazoxane, and idebenone have been used for treatment [75–77]. In doxorubicin-induced cardiomyopathy, the free-radical scavenger dexrazoxane has been shown to protect the heart from doxorubicin-associated oxidative damage, and has been recommended for clinical use to attenuate the myocardial damage that may occur in children with acute lymphoblastic leukemia treated with doxorubicin chemotherapy [78]. Both CoQ10 and idebenone have been found to markedly improve cardiac function and reduce cardiac hypertrophy in patients with FRDA [79–81]. Of interest, ataxia and other central nervous system symptoms are less affected by the administration of these antioxidants than the cardiac phenotype. In addition, idebenone seems to improve the cardiac dysfunction observed in mitochondrial cardiomyopathy [75].
CoQ10, in addition to its role as an antioxidant, also serves multiple cellular functions, including participation as an electron carrier in the respiratory chain and as an activating cofactor for mitochondrial uncoupling proteins. Furthermore, CoQ10 appears to have a beneficial effect in several neurological disorders with cardiac involvement, including MELAS and KSS syndromes [82]. Moreover, a significant reduction in the incidence of cardiac conduction abnormalities seen in patients with KSS or CPEO syndromes has been reported using CoQ10 at relatively high doses ranging from 60 to 150 mg/day [83]. Also, clinical improvement was observed in patients with advanced HF after CoQ10 supplementation to standard therapy [84]. Nonetheless, because the sample size and the design used in these studies raised concerns as to general clinical application of CoQ10 in treating HF, a large double-blind multisite clinical trial is currently underway to test its efficacy [85].
β-blocker and angiotensin-converting enzyme (ACE) inhibitor are routinely used in the management of HF. Whether carvedilol, a vasodilator β-blocker with antioxidant activity, indeed exerts beneficial antioxidant effects in patients with HF remains controversial. Whereas some investigators reported a reduction of oxidative stress in HF patients treated with carvedilol [86] others did not [87]. Using immunohistochemistry, Nakamura et al. evaluated the expression of 4-hydroxy-2-nonenal (HNE)-modified protein (a major lipid peroxidation product) in endomyocardial biopsy tissues from 23 patients with dilated cardiomyopathy (DCM) and 13 control subjects with normal cardiac function [88]. Expression of HNE-modified protein was found in all myocardial tissue samples from patients with DCM. Expression was distinctive in cardiomyocytes cytosol. Myocardial HNE-modified protein levels in patients with DCM were significantly increased compared with the levels in control subjects. In addition, biopsy samples from 11 patients with DCM were examined before and after treatment with carvedilol (5–30 mg/day; mean dosage, 22 ± 8 mg/day). Following treatment, myocardial HNE-modified protein levels decreased by 40% and with improvement in HF. Taken together, this study confirmed that oxidative stress is elevated in HF and the administration of carvedilol resulted in reduction of oxidative stress and improvement in cardiac function.
These same investigators have also examined whether levels of 8-hydroxy-2-deoxyguanosine (8-OHdG), a marker of oxidative DNA damage, were elevated in the serum and myocardium of patients with DCM, and whether carvedilol could decrease 8-OHdG [89]. Serum 8-OHdG was measured by enzyme immunoassay in 56 patients with DCM and in 20 control subjects. DCM patients had significantly increased levels of 8-OHdG compared to control subjects. Interestingly, immunohistochemically positive 8-OHdG staining was detected in the nuclei of cardiomyocytes from DCM endomyocardial tissue patients, but not in controls. After treatment with carvedilol, the serum levels of 8-OHdG in DCM patients decreased by 19%, together with improvement in HF. Thus, levels of 8-OHdG are elevated in serum and myocardium of HF patients and carvedilol seems to be an effective way to reduce oxidative DNA damage.
The antioxidative properties of β-blockers and ACE inhibitors in severe HF have been studied by Chin et al. [90]. From a group of 66 outpatients with HF, 46 patients who were on an established treatment with ACE inhibitors were started on β-blockers, and 20 patients not previously on ACE-inhibitors were treated with lisinopril. Baseline parameters were compared to 22 healthy control subjects. Serum lipid hydroperoxides (LHP) and total antioxidant capacity (TAC) were determined as indices of oxidative damage and antioxidant defense, and plasma von Willebrand factor (vWf) as an index of endothelial damage/dysfunction. The baseline indices for the measures of oxidative damage and endothelial function in the 66 HF patients were significantly higher than healthy control subjects. After 3 months of maintenance therapy with β-blockers, a significant reduction in LHP levels occurred, but not in TAC or vWf. ACE inhibitor therapy also significantly reduced vWf levels, but did not have any statistically significant effects on LHP or TAC. These findings suggest that oxidative stress in advanced severe HF may be due to increased free radical production or inefficient free radical clearance by scavengers and that β-blockers, but not ACE inhibitors, reduced lipid peroxidation (although no relation was found between a reduction in oxidative damage and endothelial damage/dysfunction).
Treatment with ACE inhibitors, angiotensin, aldosterone, and endothelin receptor blockers has been shown to beneficially modulate endothelial dysfunction in severe HF. As pointed out by Bauersachs and Widder [91], these therapies increase NO bioactivity by either modulation of ROS generation, thereby preventing the interaction of superoxide anions with NO, and/or by increasing eNOS expression/activity. In experiments in rats with large MI, treatment with AVE9488, a novel eNOS transcription enhancer, attenuates cardiac remodeling and endothelial dysfunction. Furthermore, antioxidants, l-arginine, cofactors of endothelial NO-synthase, and exercise training positively modulate endothelial function [92].
Conclusions
Molecular oxygen is central in both the formation of NO, a primary determinant of both vascular tone and cardiac contractility and in the generation of ROS, as a significant by-product of energy metabolism during its sequential acceptance of electrons in the mitochondrial ETC. These short-lived intermediates can either act as an important signaling molecule or induce irreversible oxidative damage to proteins, lipids, and nucleic acids, therefore ROS and oxygen exert both beneficial and deleterious effects. ROS play an integral role not only in the genesis of CAD but also in its progression. Several in vitro and animal studies have demonstrated that in the failing heart, ROS influence several components of the cardiac phenotype and cardiac remodeling, including contractile function, interstitial fibrosis, endothelial dysfunction, and myocyte hypertrophy. Furthermore, ROS contribute to the remodeling processes in a number of ways including activation of MMPs that participate in reconfiguration of the ECM, acting as signaling molecules in the development of compensatory hypertrophy, and contributing to myocyte loss via apoptosis signaling. ROS can be generated in the heart and endothelial tissues by nonmitochondrial reactions, including the involvement of xanthine oxidase (XO), NAD(P)H oxidases, and cytochrome p450. In addition, increased ROS and toxic oxygen metabolite production in both the myocardial mitochondrial organelle and leukocytes are exacerbated by readmission of oxygen during postischemic reperfusion.
Oxidative stress, resulting from both increased ROS generation and diminished antioxidant protection promotes the oxidation of thiol groups in proteins and lipid peroxidation leading initially to reversible damage, and finally to necrosis. Reduced bioavailability of NO and increased generation of ROS within the vascular wall are key determinants in endothelial dysfunction, and this imbalance between NO and ROS mainly results from neurohumoral activation associated with HF. Furthermore, the activation of the RAAS may play a central role. Although the use of scavengers to treat oxidative stress has been considered for quite some time, the success of this approach has been questionable at least. Nonetheless, there is increasing evidence that HF generation of ROS/RNS may be ameliorated by targeting ROS/RNS-generating enzymes and upstream mediators. β-blockers, but not necessarily ACE inhibitors, appear to reduce lipid peroxidation although so far no relation has been demonstrated between a reduction in oxidative damage and endothelial damage/dysfunction. Nevertheless, therapies that ameliorate endothelial function in heart failure have been shown to improve exercise tolerance and outcomes making endothelial dysfunction an important target to develop novel therapies based on the concepts reviewed in this chapter.
References
1.
2.
Becker LB, vanden Hoek TL, Shao ZH, Li CQ, Schumacker PT. Generation of superoxide in cardiomyocytes during ischemia before reperfusion. Am J Physiol 1999;277:H2240–H2246.PubMed
3.
Becker LB. New concepts in reactive oxygen species and cardiovascular reperfusion physiology. Cardiovasc Res 2004;61:461–470.PubMedCrossRef
< div class='tao-gold-member'>
Only gold members can continue reading. Log In or Register a > to continue
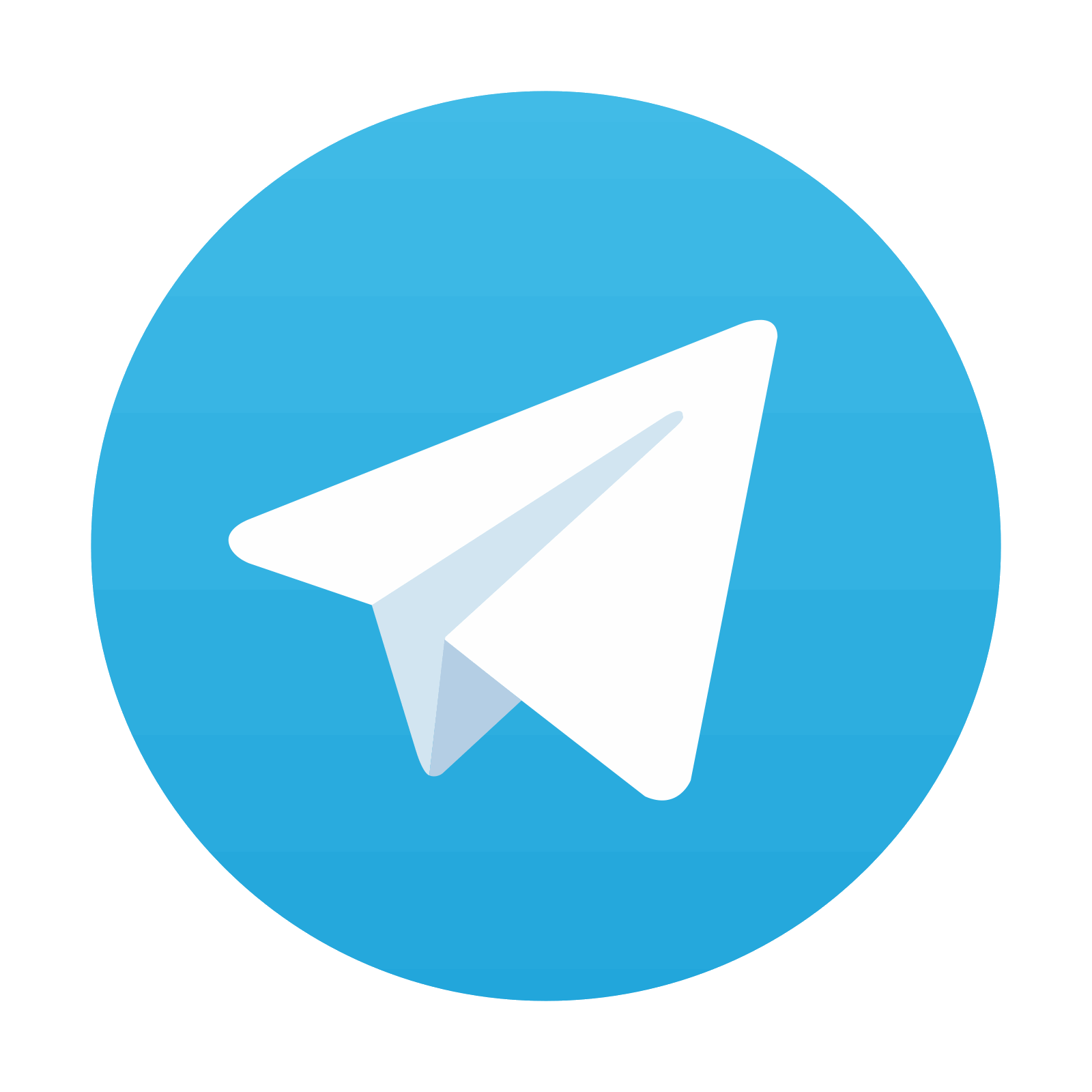
Stay updated, free articles. Join our Telegram channel
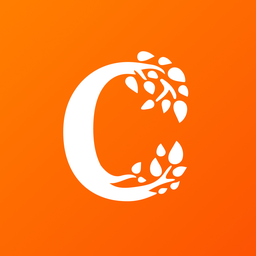
Full access? Get Clinical Tree
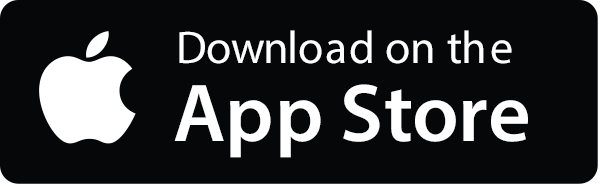
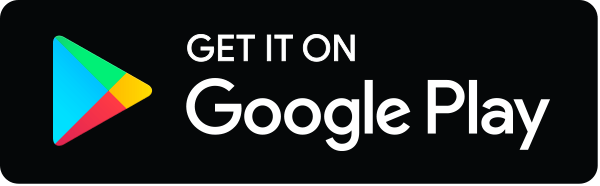