Fig. 1.
Model of the locomotor system in the lamprey. Neuronal oscillators are distributed along the spinal cord and are coupled by a coordinating system to form central pattern generator (CPG) networks. The spinal CPG networks produce the basic motor pattern for locomotion such that motoneurons (MNs) activate left and right axial muscles to contract in the proper temporal sequence. Reticulospinal (RS) neurons in a brain command system activate the spinal CPGs and initiate locomotion. Following a rostral spinal transection (T), RS neurons are disconnected from spinal CPGs, resulting in paralysis below the lesion. In the lamprey, the axons of RS neurons regenerate through the healed spinal lesion site and make synapses with spinal neurons, resulting in recovery of locomotor behavior in a few weeks (modified from (12)).
Severe SCI, such as a substantial spinal contusion or complete spinal transection, disrupts axons from descending brain neurons, including RS neurons, and results in paralysis caudal to the lesion. Also, transmission of ascending sensory information and ANS functions are compromised, but these will not be the focus of the present review. Following a complete rostral SCI, mature “higher” vertebrates, such as birds and mammals, do not behaviorally recover because of little or no axonal regeneration within the CNS (6–11), and for humans, this debilitating condition is a major health problem (6, 9). For mature higher vertebrates after neural injury, the CNS is a restrictive environment for axonal regeneration, mainly because of several proteins associated with myelin (e.g., NOGO, MAG, OMgp) and factors in the glial scar (e.g., CSPGs) that inhibit neural outgrowth (6, 9–11). In addition, neuronal cell death due to secondary injury effects (e.g., hemorrhagic necrosis, inflammation, swelling, release of neurotoxic agents) and delayed demyelination can compromise CNS functions (9), sometimes to a greater extent than the primary effects of the injury.
In contrast, following spinal cord transections in “lower” vertebrates, such as lamprey, fish, and certain amphibians, there is robust axonal regeneration through the healed spinal lesion site and, remarkably, virtually complete behavioral recovery in a few weeks (reviewed in (12–14)). For example, after SCI in these lower vertebrates, injured axons regenerate through the lesion site, sometimes over relatively long distances (15, 16), usually in the correct directions and often in the correct spinal tracts ((17–19); however see (20)), and form synaptic connections with appropriate spinal targets on the opposite side of the lesion site (21, 22).
2 Why Does the Lamprey Recover Locomotion Following SCI?
Following a complete spinal transection in the lamprey, ependymal cells proliferate and form a bridge between the cut ends of the spinal cord (reviewed in (12, 13)). Transected ascending and descending axons form growth cones and regenerate along the ependymal bridge across the injury site. Regenerating axons form synapses on the other side of a healed spinal lesion site, and behavioral functions, such as locomotion, begin to recover. With increasing recovery times, greater numbers of axons extend across a healed transection site and for increasing distances, and within a few weeks there is virtually complete recovery of behavioral functions. However, the capacity for functional axonal regeneration in the CNS of lampreys and other lower vertebrates probably has not been selected for evolutionarily but appears to be a byproduct of other factors or conditions (see below).
There are a number of possible reasons why functional axonal regeneration occurs in the lamprey and certain other lower vertebrates (reviewed in (12, 13)). First, in lower vertebrates, new neurons are added to the CNS, albeit slowly, well after the main phase of neural development (23, 24) as a result of delayed maturation of neuronal precursors (25) and/or neurogenesis (26) (see (27)). Persistent neurodevelopmental mechanisms allow newly added neurons to send out axons and make synapses with appropriate targets. Thus, the permissive conditions and guidance cues that regulate these functions for newly added neurons probably also support axonal regeneration and synaptic reconnection for injured neurons following SCI (13, 22). However, for the lamprey (28, 29) and several other lower vertebrates ((30); also see (13)), axonal regeneration, and not the addition of new neurons, is the main mechanism for restoration of brain–spinal cord projections and recovery of locomotor function after SCI. Second, in lower vertebrates, myelin either is absent ((31), lamprey) or, if present, appears to lack sufficient quantities of the inhibitory proteins that suppress axonal regeneration in higher vertebrates (32). Third, in lower vertebrates, the spinal cord glial scar is not an impenetrable barrier to axonal regeneration (17), and “secondary injury” effects appear to be minimal or nonexistent. Fourth, lower vertebrates are cold-blooded, and their relatively low metabolism and low oxygen needs make them partially resistant to mild anoxia that might result from disruption of blood vessels following SCI. In mammals, including humans, modest hypothermia has a neuroprotective effect following SCI (33, 34) due, in part, to lowering the metabolism of neural tissue in the vicinity of the injury site.
3 Lamprey Species Used for SCI Research
Lampreys are jawless fish in the class Agnatha (sometimes called cyclostomes), which are thought to have appeared ∼390–470 million years ago (35), and are considered a key primordial species in the evolution of vertebrates. All of the ∼40 living species of lamprey have an initial larval phase (ammocoete), which lasts several years and is characterized by burrowing in the mud of streams or rivers and filter feeding on microorganisms (35). Subsequently, larval animals metamorphose into adults, and for about half of the lamprey species the adults migrate downstream into lakes or the ocean to become parasitic feeders on fish (35). After a few years and substantial growth, the parasitic adults migrate upstream, spawn, and die.
Larval sea lampreys (Petromyzon marinus) have been used for almost all of the lamprey studies on SCI and neural regeneration (13, 14). Relatively large numbers of these animals can be collected easily and then maintained in the laboratory for moderately long recovery times following SCI. Neural regeneration and behavioral recovery also occur following SCI in young adult sea lampreys (36). However, because animals at this life stage are parasitic feeders, maintenance of paralyzed or partially paralyzed spinal cord-injured adult animals in the laboratory for long recovery times can be problematic.
4 Conceptual and Biological Framework for Using the Lamprey
Following severe SCI in mature higher vertebrates, axonal regeneration normally is very limited, and there is little or no behavioral recovery. Thus, at present in these animals it is not possible to study the full complement of mechanisms that might be necessary for substantial axonal outgrowth and restoration of locomotor functions. In contrast, the lamprey and certain other lower vertebrates offer the unique opportunity to identify the sets of conditions that support unaided neural repair following SCI and virtually complete recovery of locomotor behavior. Thus, these lower vertebrates can provide a set of target goals for the types of cellular conditions that are required to promote substantial axonal regeneration and recovery of behavioral functions following SCI. These conditions, if created in mammals following SCI, presumably would support axonal regeneration and result in behavioral recovery. Finally, even though the lamprey CNS is permissive for axonal outgrowth, in this animal neural regeneration following SCI has some limitations (see Sect. 6.2.3). These limitations provide insights into potential problems that might occur in the future if the mammalian CNS can be modified to be substantially permissive for axonal regeneration, perhaps similar to the conditions in the lamprey nervous system.
5 Rationale for Using the Lamprey for SCI Research
5.1 Advantages
The lamprey has a number of unique and powerful advantages that make it ideally suited for determining the mechanisms responsible for neural regeneration following injuries of the spinal cord (reviewed in (12–14)): (a) The lamprey CNS can repair itself after SCI, and this results in virtually complete behavioral recovery in a few weeks (Fig. 2) ((13); also see (15, 28, 37, 38)). (b) The movements and motor activity during locomotion (swimming) are relatively simple and easy to analyze which allows reliable assessment of locomotor function at various recovery times after SCI (Figs. 2 and 3a). Furthermore, locomotor networks can be studied using in vitro CNS preparations (Fig. 3b) (38–40), which allow control of the ionic and pharmacological makeup of the bath, elimination of mechanosensory inputs, and mechanically stable conditions for intracellular recordings. (c) The lamprey CNS has many basic features in common with the nervous systems of higher vertebrates (Fig. 4a) (41, 42), but because of its primitive nature, it is comparatively simple. As such, in spinal cord-transected lamprey, cellular, synaptic, and integrative properties of brain and spinal cord locomotor networks are more easily analyzed than those in higher vertebrates. Of particular importance, the neural circuits in the brain and spinal cord that control locomotor behavior in normal, uninjured lampreys have been intensely studied (43, 44), and there is a wealth of information that can be translated to the study of SCI using this animal. (d) Several classes of large, identifiable neurons in the lamprey brain (45) and spinal cord (44, 46) can be investigated from one animal to the next and are very useful for certain types of studies. For example, the lamprey brain contains ∼28 large, uniquely identified RS neurons called Müller and Mauthner cells (Fig. 4b) (45). In addition, the brains of larval lampreys also contain over 1,000 smaller, unidentified RS neurons that can be investigated with neurophysiological and anatomical techniques (47, 48). (e) In larval lamprey, the thin CNS (∼100–400 μm thick) permits imaging in live or fixed whole-mount tissue (Fig. 4c), which preserves the 3D aspects of the nervous system. (f) The lamprey genome has recently been characterized (http://pre.ensembl.org/Petromyzon_marinus/index.html), and the lamprey has the potential to become a new model system for molecular neurobiology. (g) Following SCI, axonal regeneration of RS neurons is critical for restoring locomotor function. At present, the lamprey is the only animal in which it is feasible to study all of the following features of these important neurons: electrophysiological properties (47, 48); synaptic physiology and network function (43, 44, 49); synaptic structure (50, 51); immunohistochemistry (49); gene expression (48, 52); neuroanatomical projections (15, 16); neuron morphology (29); in vivo imaging (53); intracellular calcium dynamics (54); neurite outgrowth in cell culture (55); and neural regeneration following SCI (reviewed in (12, 13)).
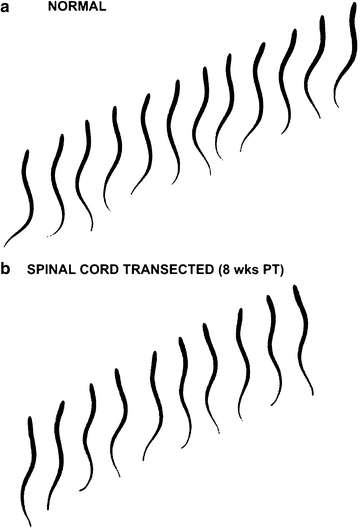
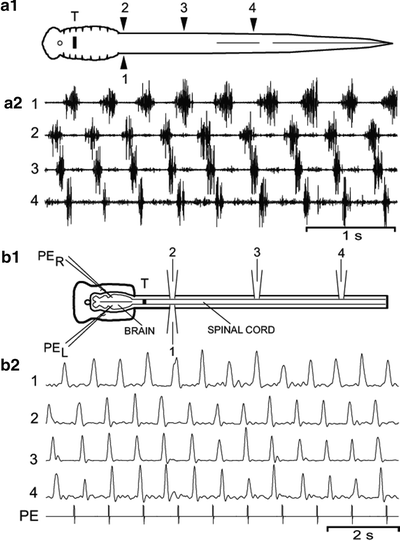
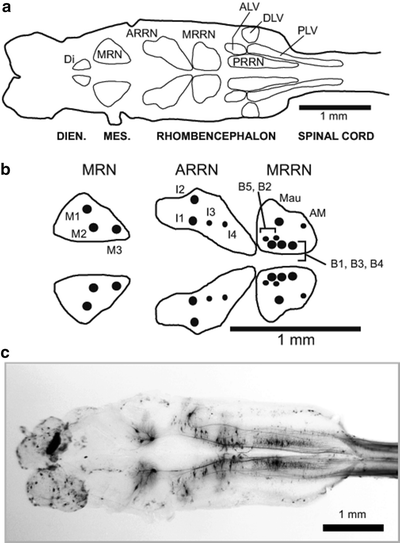
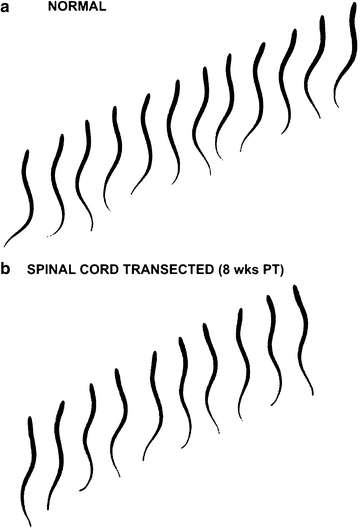
Fig. 2.
(a) Sequential video images, left-to-right, during swimming movements in a normal larval sea lamprey. Swimming movements are characterized by left–right bending at each level of the body, and S-shaped body undulations that propagate toward the tail and propel the animal forward. (b) Swimming movements in an animal at 8 weeks following a complete spinal transection at 10% BL. Interframe interval = 133 ms for both sequences.
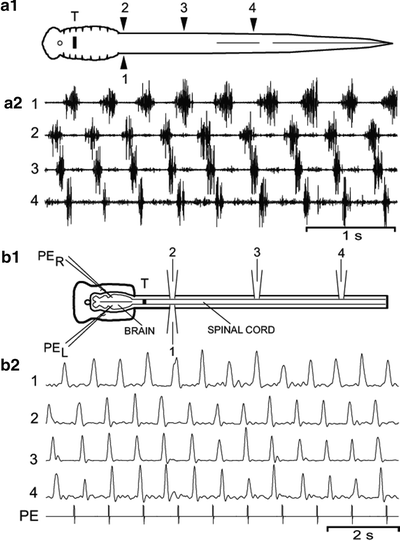
Fig. 3.
(a) Swimming muscle burst activity in whole animals. (a1) Diagram of a larval lamprey that recovered for 8 weeks following a spinal transection (T) at 10% BL. Muscle recording electrodes are located at 20% BL (electrodes 1,2), 40% BL (3), and 60% BL (4). (a2) Muscle burst activity during swimming consisting of left–right alternation (1 ↔ 2) and rostrocaudal phase lags (2 → 3 and 3 → 4). (b) Ventral root bursts during in vitro swimming activity. (b1) Diagram of an in vitro brain–spinal cord preparation from a larval animal that recovered for 32 weeks following a spinal transection (T) at 10% BL. Swimming activity is initiated by pharmacological microstimulation (PER, PEL) in brain locomotor areas and recorded from spinal ventral roots at 20% BL (electrodes 1,2), 40% BL (3), and 60% BL (4). (b2) Focal pressure ejection (PE) of excitatory agents in brain locomotor areas initiates locomotor ventral root burst activity (1–4; full-wave rectified and integrated, τ = 50 ms) consisting of left–right alternation (1 ↔ 2) and rostrocaudal phase lags (2 → 3 and 3 → 4) (modified from (37, 38)).
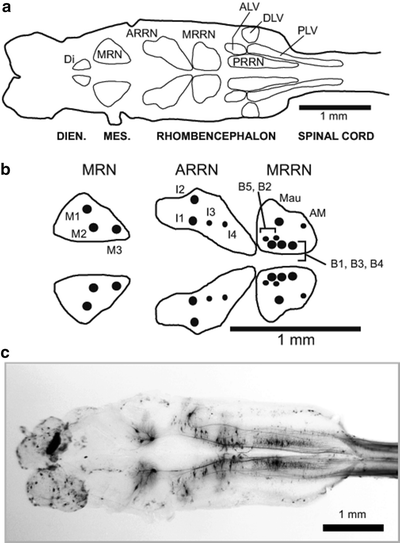
Fig. 4.
(a) Diagram of a larval lamprey brain (left) and rostral spinal cord (right) showing contours around the main groups of descending brain neurons (DIEN. = diencephalon, MES. = mesencephalon). Reticular cell groups: MRN—mesencephalic reticular nucleus; ARRN (anterior), MRRN (middle), and PRRN (posterior) rhombencephalic reticular nuclei. Non-reticular cell groups: Di—diencephalic; ALV—anterolateral vagal; DLV—dorsolateral vagal; and PLV—posterolateral vagal. (b) Enlargement of right (top) and left (bottom) reticular nuclei that contain large, uniquely identified RS neurons (Müller cells): M cells (M1–M3) in the MRN, I cells (I1–I4) in the ARRN; and B cells (B1–B5) in the MRRN. Mauthner (Mau) and auxiliary Mauthner (AM) cells are additional identified RS neurons located in the MRRN. Note that large, identified RS neurons in the MRN, ARRN, and MRRN are intermingled with smaller, unidentified RS neurons, which are omitted for simplicity. (c) Photomicrograph of a larval lamprey brain showing descending brain neurons in reticular and non-reticular nuclei that were retrogradely labeled by application of HRP to the spinal cord at 20% BL (modified from (15, 16)).
5.2 Disadvantages
The larval phase and often the adult phase of lampreys last several years, it is difficult to raise lampreys in the laboratory for long periods of time, and these animals have not been bred in captivity. Therefore, the types of genetic techniques that can routinely be performed with Drosophila, zebrafish, and mice are not available for SCI experiments using lampreys.
Although the permissive conditions for neurite outgrowth in the lamprey CNS are an obvious advantage for certain types of studies (see Sect. 5.1 above), this situation also precludes testing possible manipulations for overcoming the specific mechanisms that inhibit axonal regeneration within the CNS of higher vertebrates. Thus, for SCI studies, the lamprey is mainly useful for determining the sets of conditions that permit functional axonal regeneration and result in behavioral recovery, rather than the mechanisms that hinder neuronal outgrowth in higher vertebrates.
6 Recovery of Locomotor Initiation: Descending Projections
6.1 Lesion Types and Sites, and Survival Times
For lamprey studies, SCIs typically involve complete spinal cord transections to ensure that all descending and ascending axons are severed at the injury site, and that any residual behavioral functions are not due to spared projections. To investigate the restoration of descending brain–spinal cord projections and recovery of initiation of locomotor behavior following SCI, complete transections usually are performed at very rostral spinal levels to disrupt all descending drive from brain locomotor command systems to spinal CPG networks (T in Fig. 1). For example, in studies from the author’s laboratory, SCIs in larval sea lamprey (∼100 mm typical length) often are performed at 10% body length (BL, normalized distance from anterior tip of head; brain/spinal cord junction ∼8% BL), which is located ∼2–3 mm below the brain (15, 28, 37, 38). For all surgical procedures, animals are anesthetized in ∼100–200 mg/l tricaine methanesulfonate (MS222; Crescent Research Chemicals; Phoenix, AZ USA), which is absorbed via the gills and is a standard anesthetic for fish. The rostral spinal cord is exposed through a ∼5–10 mm dorsal incision, and small custom retractor hooks fashioned from suture needles hold the incision open to allow good visibility and free use of the hands. With fine iridectomy scissors, the exposed spinal cord is completely transected, and the completeness of the lesion is confirmed by gently lifting the meninges on one side of the transection to briefly expose the cut, cross-sectional surface of the cord. The incision is manually closed, and animals are returned to their home aquaria to recover for 2–32 weeks at ∼23°C. Initially, animals with very rostral spinal transections are paralyzed and unable to swim, and subsequently locomotor behavior gradually recovers as descending axons from brain neurons regenerate through the healed lesion site and reconnect with spinal CPGs (see Sects. 6.2.1, 6.2.2, and 6.2.3). For the lamprey, almost all of the SCI studies described in this review have been carried out at approximately room temperature (∼23°C), and at colder temperatures (e.g., 12°C), axonal regeneration is depressed and behavioral recovery is substantially delayed (56).
6.2 Outcome Measures and Key Findings
6.2.1 Kinematic Analysis and Locomotor Muscle Burst Activity in Whole Animals
Following rostral spinal cord transections in larval lampreys, behavioral recovery has been assessed using two main techniques. First, swimming movements, from their earliest appearance following SCI until they approach those of normal animals, have been quantified using kinematic analysis. For example, swimming in normal larval lampreys is characterized by two main features (Fig. 2a) (37): (a) left-right bending of the body at each segmental level; and (b) S-shaped body undulations that propagate toward the tail with increasing lateral amplitude to push against the water and propel the animal forward. Behavioral responses are elicited by brief electrical stimulation of the head or tail (1–10 mA, 2 ms pulses at 100 Hz for 50 ms), and swimming movements are captured with a high-resolution recording system (e.g., 30 frames per second, ∼4–8 ms shutter speed). Individual frames are displayed on a computer screen, at least 11 x,y points are digitized along the midline of the body, and these coordinates are used to calculate several features of swimming behavior: cycle times (T) or frequencies (f = 1/T) of rhythmic swimming movements; amplitudes of lateral displacement (i.e., left-right bending) versus distance along the body; and mechanical wavelength (λ), which is the length of an S-wave along the body divided by total body length. These parameters for swimming in control lampreys have been compared statistically to those in experimental animals at various recovery times following rostral spinal transections.
Second, for partially recovered spinal cord-injured lampreys, active muscle contractions in the rostral body generate undulatory swimming movements that can passively propagate to the caudal body and produce swimming-like movements, even though muscles in the lower body are inactive (37, 57). Thus, at various recovery times following spinal cord transections it is important to record axial muscle activity (EMGs) during swimming to determine the actual degree of behavioral recovery. For larval lampreys, muscle recording electrodes are fashioned by using a 30 Ga. needle to separately insert two fine wires (56 μm dia.), insulated except at the tip, into musculature at various points along the body (electrodes 1–4; Fig. 3a1). All of the EMG wires are grouped in a bundle and sutured to the body just behind the head, and the wire bundle is led to a light-weight “boom arm” so animals can swim freely in a tank (22 cm × 40 cm with ∼2–6 cm of water). Muscle activity during swimming is characterized by two features (Fig. 3a2) (37): (a) left–right alternation of burst activity at each level of the body (electrodes: 1 ↔ 2); and (b) a rostrocaudal delay of ipsilateral burst activity (2 → 3, 3 → 4). During neurophysiology experiments, raw muscle burst activity is amplified (×1,000), filtered (100 Hz to 5 kHz), and stored on tape (Neuro-Data DR890, 11 kHz sampling rate per channel; Cygnus Technologies, Delaware Water Gap, PA USA), and subsequently the data are acquired using a custom eight-channel data acquisition-analysis system (DT-3016 data acquisition board; Data Translation, Marlboro, MA USA). The onset and offset times of locomotor muscle bursts are marked with the data acquisition system, and these values are imported into a spreadsheet to calculate various parameters of swimming muscle burst activity (see (37, 57–59) for definitions): cycle times (T); burst proportions (BP); intersegmental phase lags (ΦINT); and right–left phase values (ΦRT-LT). These parameters for swimming in control lampreys have been compared to those in experimental animals at different recovery times following rostral spinal cord transections.
Immediately after transection of the rostral spinal cord, lampreys are paralyzed, but at about 2 weeks post-transection (PT), weak undulatory movements and low-amplitude muscle burst activity begin to appear just caudal to the healed spinal lesion site (37). Between 2 and 8 weeks PT, left–right alternating locomotor movements gradually increase in amplitude (see Fig. 2 in (12)) and, remarkably, by 8 weeks PT are not significantly different from those of normal animals (Fig. 2b) (37). At 10–12 weeks PT, mechanical wavelengths (λ) during swimming are very similar to those for normal animals (51). In addition, with increasing recovery times, muscle burst activity occurs at progressively greater distances caudal to the spinal transection site ((37); see Fig. 3 in (12)), so that the general pattern of locomotor activity, with very minor exceptions, returns to normal by 8 weeks PT (Fig. 3a, see “muscle” in Fig. 5d).
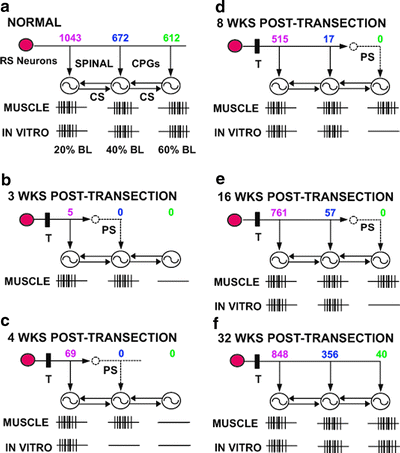
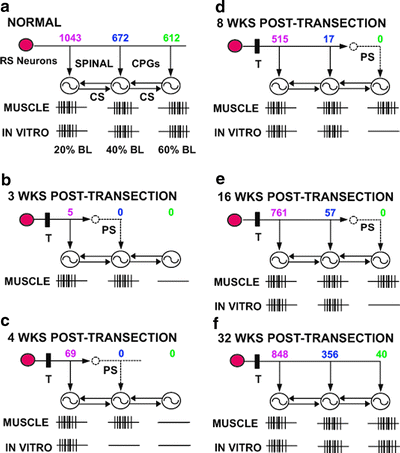
Fig. 5.
Summary of locomotor systems in normal lamprey and spinal cord-transected animals. Reticulospinal (RS) neurons in the brain activate spinal oscillators (circles with oscillator symbols), which are coupled by a spinal coordinating system (CS). Values above each oscillator are the numbers of RS neurons that project to 20, 40, and 60% BL. “MUSCLE” is locomotor muscle burst activity from whole animals (see Fig. 3a), and “IN VITRO” is locomotor ventral root burst activity from in vitro brain–spinal cord preparations (see Fig. 3b). Healed spinal cord transection sites (T) are at 10% BL. (a) In normal animals, RS neurons directly activate the spinal locomotor networks at 20, 40, and 60% BL. (b–f). Between 3 and 32 weeks PT, locomotor muscle burst activity and ventral root burst activity occur for progressively greater distances along the body below the transection site (T), but with different time courses (see text). Concurrently, RS neurons regenerate their axons for increasingly greater distances below the healed transection site. At early and intermediate recovery times, descending propriospinal (PS) neurons relay descending drive from RS neurons to lower levels of the cord that have not yet received direct input from the brain. At long recovery times, descending PS neurons are in parallel with direct descending pathways from the brain and probably supplement descending drive (not shown; see Fig. 7) (modified from (12)).
6.2.2 Locomotor Activity from In Vitro Brain–Spinal Cord Preparations
In whole animals with rostral SCIs at 10% BL, CNS neural networks as well as peripheral mechanosensory feedback can contribute to the initiation and generation of swimming muscle activity. To determine the contributions of central mechanisms alone, brain-initiated locomotor burst activity has been recorded from in vitro brain–spinal cord preparations (Fig. 3b1), in which sensory feedback is blocked. After removing the skin, musculature, and internal organs along the body, and exposing the dorsal surface of the brain and spinal cord, the preparation is transferred to a recording chamber containing lamprey Ringer’s solution (38) maintained at 6–8°C. Also, 15 mg/l d-tubocurarine chloride (Sigma Chemical; St. Louis, MO, USA) is added to the bath to block contractions of remaining body musculature and eliminate movement-related sensory feedback. The chamber is cooled with thermoelectric modules, but refrigerated circulators also are commonly used. With a 0.1 mm dia. stainless steel pin, ventral roots are separated from connective tissue along the lateral edges of the spinal cord. The tips of glass suction electrodes are placed in contact with exposed ventral roots to record locomotor burst activity, which is amplified (×1,000), filtered (10 Hz to 2 kHz), and full-wave rectified/integrated (τ = 50 ms) to better reveal the onsets and offsets of burst activity.
For in vitro brain–spinal cord preparations, spinal locomotor activity is initiated by pharmacological microstimulation in brain locomotor areas (49, 60, 61). Two micropipettes are filled with 5 mM d-glutamate/5 mM d-aspartate in Ringer’s solution (pH 7.4), and Fast green is added to visualize the ejection bolus (38, 58, 62). The micropipette tips are inserted into the bath, and while applying pressure ejection pulses (5–10 ms at 1 Hz; 20 psi), the tips are broken off to ∼2–5 μm with fine forceps. Typically, each pressure ejection pulse releases a bolus with a diameter of ∼25–50 μm, which is equivalent to ∼8–65 pl (61). The tips of the micropipettes are then inserted bilaterally ∼25–50 μm below the dorsal surface of the brain in locomotor areas, and excitatory agents are pressure ejected into these areas to activate nearby neurons. This stimulation directly or indirectly activates brain locomotor command systems, which project to and excite spinal CPGs to initiate locomotor burst activity (49, 60). At the end of a stimulation sequence (usually ≤1 min), the diameter of the ejection area within the tissue stained with Fast Green is less than ∼100–150 μm (width of brain ∼1.0–1.5 mm). Following each stimulation sequence a “rest” period of at least 3 min is allowed to reduce fatigue, during which the preparation should be inactive, before stimulation is performed again in the same locomotor area (62). To mark stimulation sites for later determination of their precise locations, the tip of a separate micropipette containing 1–3% Alcian Blue in Ringer’s solution is positioned at a stimulation site, and a small amount of the dye is pressure ejected (60, 61). Alcian Blue does not appear to compromise the viability or responsiveness of in vitro preparations during subsequent stimulation trials and remains visible after histological processing.
In vitro brain–spinal cord preparations have been set up from control larval lamprey as well as from experimental animals at 4–32-week recovery times following rostral SCI (38). For control animals, stimulation in brain locomotor areas initiates ventral root burst activity at 20, 40, and 60% BL that is characteristic of locomotor behavior (see “in vitro” in Fig. 5a). For experimental animals (4–32 weeks PT), locomotor burst activity occurs at progressively greater distances caudal to a healed spinal transection site with increasing recovery times ((38); see Fig. 4 in (12)) and gradually returns to normal (Figs. 3b and 5b–f). However, the rate at which this in vitro ventral root activity recovers is much slower than that for EMG activity from spinal cord-transected whole animals. Specifically, for experimental whole animals the pattern of locomotor muscle activity returns to normal by ∼8 weeks PT (Figs. 3a and 5d), but for in vitro preparations a normal pattern of locomotor ventral root activity is not present until 24–32 weeks PT (Figs. 3b and 5f). Much of this difference probably is due to the absence of sensory feedback and lower CNS excitability for in vitro brain–spinal cord preparations compared to whole animals. Thus, although the degree of behavioral recovery is dependent to a large extent on axonal regeneration, other mechanisms, such as mechanosensory feedback (see Sect. 9.2.1) and various compensatory mechanisms (see Sect. 6.2.4), also contribute significantly (12, 13, 38, 63). Finally, at 32 weeks PT, regenerated axons from RS neurons can directly activate the spinal CPG networks in the caudal cord under conditions in which conduction via descending propriospinal relay pathways is locally blocked in the rostral and middle spinal cord with a low-calcium Ringer’s solution (Fig. 5f; see Fig. 6 in (38))
6.2.3 Axonal Regeneration of Descending Brain Neurons
At different recovery times (3–32 weeks) following spinal transections at 10% BL, the time course of restoration of brain–spinal cord projections has been determined by retrograde labeling of descending brain neurons with horseradish peroxidase (HRP) applied to the spinal cord at 20, 40, or 60% BL. These data have been compared to those for control animals in which tracer is applied to the same levels of the spinal cord. For larval sea lamprey, descending brain neurons are located in eight main cell groups in the brain (Fig. 4a, c), and ∼80% of these cells are moderately small, unidentified RS neurons (15). In addition, moderately small RS neurons are both necessary (49) and sufficient (61) for initiating spinal locomotor activity, and these particular neurons are located in four reticular nuclei (Fig. 4a) (41, 42, 45): mesencephalic reticular nucleus (MRN); and the anterior (ARRN), middle (MRRN), and posterior (PRRN) rhombencephalic reticular nuclei.
Through a dorsal incision centered at 20, 40, or 60% BL, the spinal cord is exposed and transected with fine iridectomy scissors. A small piece of Gelfoam (∼1 mm3; Upjohn; Kalamazoo, MI, USA) soaked in a solution of 40% HRP (type VI, Sigma Chemical, St. Louis, MO, USA) and 1% dimethyl sulfoxide (DMSO) in water is applied between the cut ends of the cord. The incision is closed and sealed with a thin line of cyanoacrylate adhesive (Super Glue Gel; Loctite, Rocky Hill, CT, USA), and subsequently animals are returned to their home aquaria. After a ∼14-day HRP transport time, brains are removed, and whole-mount tissue is histologically processed in a modified Hanker-Yates solution consisting of 0.10% catechol, 0.05% phenylenediamine, and 0.0375% H2O2 in 0.1 M Tris buffer (pH 7.4) for 5–25 min at ∼0°C (15, 16, 29). Subsequently, the tissue is dehydrated in an ethanol series, cleared in methyl salicylate, and mounted on slides with Permount (Fisher Scientific, St. Louis, MO, USA). Data analysis is performed with a custom computer-microscope anatomical workstation, which includes stepper motors attached to the x and y axes of a microscope stage, stepper motor controller board, and joystick, all of which are interfaced with a custom computer program. The joystick controls the movements of the microscope stage, and a cross-hair in one of the eyepieces is first moved along the outline of the brain/spinal cord (trace mode) and then aligned with individual labeled neurons (mark mode). The x,y coordinates of the tissue outlines and labeled neurons are captured by the computer, which then tabulates the numbers of labeled neurons in different cell groups and also generates a diagram of the brain and neuronal labeling pattern, as if all structures are in the same focal plane. The numbers of labeled descending brain neurons that have extended their axons beyond the lesion site to rostral, middle, or caudal regions of the spinal cord have been compared statistically to the numbers of neurons that project to these same spinal levels for normal animals.
For normal larval lampreys, moderately large numbers of brain neurons project to the spinal cord at 20% BL (∼1,250 total descending brain neurons, ∼1,043 RS neurons), 40% BL (∼900 total neurons, ∼672 RS neurons ), or 60% BL (∼825 total neurons, ∼612 RS neurons) (Fig. 5a) (15). Thus, for normal animals, ∼60% of both descending brain neurons and RS neurons project for relatively long distances to caudal levels of the spinal cord (Fig. 6a). For spinal-transected animals, axons from descending brain neurons, including RS neurons, project for progressively greater distances caudal to a healed spinal transection site with increasing recovery times (Figs. 5b–f and 6b, c) ((15); also see (12)). However, at most recovery times, the distances that RS neurons have regenerated their axons caudal to a spinal lesion site are much less than the projection distances in normal animals. For example, at 8-week recovery times, virtually all of the regenerated axons of RS neurons project just caudal to the lesion site (Fig. 6b). At 32-week recovery times, the numbers of RS neurons that project to the rostral cord are not significantly different from those for normal animals, but projections to the middle and caudal cord are ∼50% and ∼6%, respectively, of those for normal animals (15). Thus, at this relatively long recovery time, about 60% of descending brain neurons, including RS neurons, that have regenerated their axons do so for relatively short distances to rostral levels of the spinal cord (Fig. 6c). Accordingly, even at relatively long recovery times, descending brain neurons display incomplete axonal regeneration such that the normal brain–spinal cord projection patterns are not restored (15).
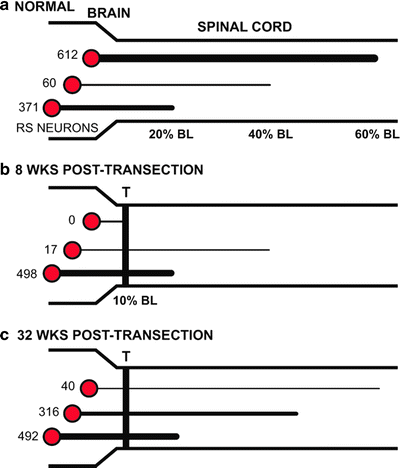
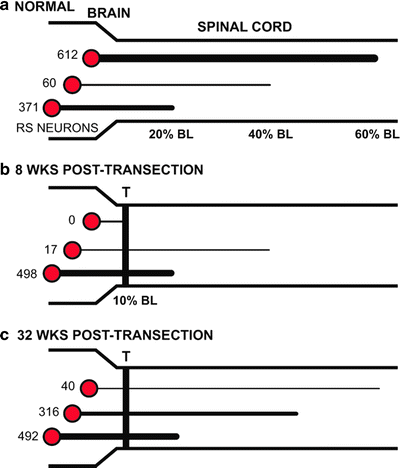
Fig. 6.
Summary of projections from RS neuron in normal and spinal cord-transected larval lamprey. The thickness of horizontal lines from RS neurons indicates the relative numbers of descending projections. (a) In normal animals, about 60% of RS neurons project for relatively long distances to at least the caudal spinal cord (60% BL). (b) At 8-week recovery times following spinal transections (T) at 10% BL, ∼97% of RS neurons that have regenerated their axons do so for only short distances into the rostral spinal cord (∼20% BL). However, at this recovery time in whole animals, locomotor muscle burst activity has returned to normal along the body (see Figs. 3a and 5d). (c) At 32-week recovery times, ∼60% of RS neurons that regenerate their axons do so for relatively short distances, only as far as the rostral spinal cord (modified from (15, 16)).
The mechanisms that limit neural outgrowth and that are responsible for incomplete axonal regeneration within a permissive CNS very likely are substantially different from those that inhibit axonal regeneration in mammals. Specifically, for the lamprey and other lower vertebrates, many regenerating axons of descending brain neurons make synapses a short distance caudal to a healed spinal lesion site, and these synaptic connections are thought to suppress or limit additional axonal extension (12, 13, 64), a phenomenon sometimes referred to as “synaptic capture” (30). For example, in the lamprey, axonal regeneration has been compared following rostral (10% BL) and caudal (50% BL) spinal cord transections (64). Rostral spinal transections, which eliminate virtually all synapses that descending brain neurons make in the spinal cord, are a much stronger stimulus for axonal regeneration than caudal transections, which preserve many of these synapses above the lesion site. We hypothesize that the synapses descending brain neurons make, either above or below a healed spinal lesion site, regulate the degree to which these neurons are stimulated to regenerate (64).
Although the projections of descending brain neurons and their connection patterns with spinal neurons below the transection site are not restored to normal even at 32 weeks after SCI, there still is virtually complete recovery of locomotor behavior in about 8 weeks (reviewed in (10, 11)). Thus, minimal or moderate axonal regeneration can result in substantial locomotor recovery, provided that certain compensatory mechanisms are available (see Sects. 6.2.4, 9.2.1, and 11). This is potentially promising information regarding human SCI because it suggests that induction of robust and/or long-distance axonal regeneration might not be necessary for mediating considerable behavioral recovery. A much more modest degree of axonal outgrowth could be sufficient, and this would place less demands on potential therapeutic interventions.
6.2.4 Compensatory Mechanisms: Descending Propriospinal Relay Neurons
Propriospinal (PS) neurons serve a number of functions within locomotor systems (reviewed in (65): (a) ascending and descending PS neurons couple spinal oscillators to coordinate locomotor activity at different levels of the body (Fig. 1; see Sect. 9.2) (3, 66); (b) ascending PS neurons transmit timing and other important feedback information to the brain to modulate the activity of neurons in several descending systems (see Sect. 10) (5); and (c) descending PS neurons relay activity from descending brain neurons to more caudal levels of the spinal cord (Fig. 7a) (63, 67). In normal larval lamprey, the distributions of descending PS relay neurons in the rostral and middle spinal cord with short-to-moderate length axons have been determined by application of HRP to the spinal cord at 40% BL or 60% BL (63). For experimental animals with rostral spinal transections at 10% BL, HRP has been applied to the same spinal levels following recovery times of 4–32 weeks.
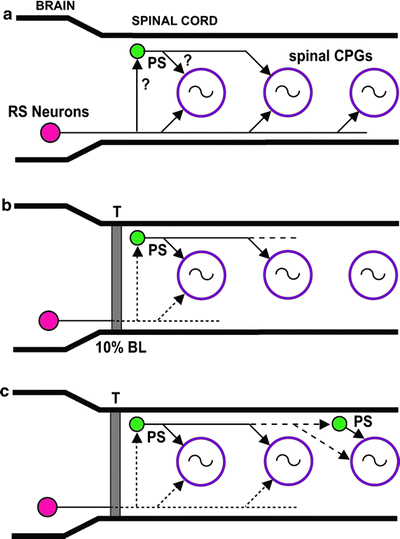
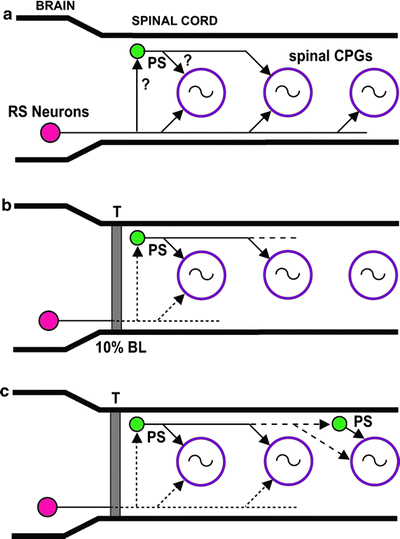
Fig. 7.
Model of possible roles of descending propriospinal (PS) relay neurons. (a) In normal larval lamprey, RS neurons directly activate spinal CPG networks (circles with oscillator symbols) all along the spinal cord. Note that spinal coordinating systems (see Fig. 1) have been omitted for simplicity. Descending PS neurons relay descending drive from RS neurons and contribute to the activation of spinal CPGs. (b) At early recovery times (3–4 weeks PT) following spinal transections at 10% BL (T), RS neurons have regenerated their axons for relatively short distances and can only directly activate spinal CPGs in the rostral spinal cord. Some PS neurons relay descending drive from brain command systems to CPG networks in the middle spinal cord (see Fig. 5b, c). (c) At intermediate-to-long recovery times (8–16 weeks PT), a few RS neurons directly activate CPGs in the rostral and middle spinal cord (see Fig. 5d, e). Descending PS neurons have sprouted their axons and can directly and/or indirectly relay descending drive to CPGs in the middle and caudal spinal cord (modified from (63)).
In normal lampreys, descending PS neurons are distributed along much of the spinal cord (63), and the majority of these neurons have relatively short axons (<10–15 mm), although a few neurons have moderate-to-long axons (∼25–50 mm). Following rostral SCI, it is very likely that some of these neurons relay descending drive from RS neurons to lower levels of the spinal cord (Figs. 5 and 7b). For example, at early recovery times, PS neurons can substitute for the absence of direct projections from RS neurons to middle and caudal levels of the spinal cord (Fig. 5b–e). At longer recovery times, PS neurons are in parallel with and probably supplement the effects of the relatively few direct descending projections from RS neurons to these spinal levels (Fig. 7b) (see Fig. 7 in (38); reviewed in (12, 13)). Although neuronal outgrowth of RS neurons contributes substantially to behavioral recovery following SCI, descending PS neurons represent a very important compensatory mechanism that can make up for incomplete axonal regeneration of these descending brain neurons. Interestingly, studies with neonatal rats indicate that when long, direct brain–spinal pathways are disrupted, polysynaptic pathways via descending PS neurons can relay descending drive from the brain to caudal CPG networks and initiate locomotor activity (68). Thus, descending PS neurons represent a potentially important target for therapeutic interventions following SCI (65, 67).
For larval lamprey following spinal transection at 10% BL, significantly greater numbers of uninjured descending PS neurons below the lesion site project to more caudal spinal levels than in control animals (63). This plasticity very likely is due to descending PS neurons that have sprouted and extended their axons to more caudal levels of the spinal cord (Fig. 7b), possibly because of being disconnected from brain centers and/or factors released from other spinal targets below lesion sites (see (69)). Plasticity of descending PS neurons below a spinal injury site would be expected to enhance the compensatory functions of these neurons.
6.2.5 Axonal Regeneration Versus Neural Developmental Mechanisms
In principle, following SCI the restoration of descending projections of RS neurons might be due to axonal regeneration, which requires that the axons of these neurons (a) are injured and (b) subsequently extend across the healed spinal lesion site. Alternatively, some axonal growth across a spinal lesion site might be due to developmental mechanisms involving uninjured RS neurons, either ones with relatively short axons that were not lesioned by SCI or new neurons that have been added to the nervous system. At present, axonal regeneration appears to be one of the most attractive potential mechanisms for repair following mammalian SCI, and therefore, if the lamprey is to serve as a model system, it is important to demonstrate that axonal elongation is largely due to this mechanism and not development.
In the lamprey brain, Müller and Mauthner cells are large, uniquely identifiable RS neurons that project for virtually the entire length of the spinal cord (42, 45). These large RS neurons, which are intermingled with smaller, unidentified RS neurons, are located in three reticular nuclei (Fig. 4b): M cells (M1–M3) in the MRN; I cells (I1–I4) in the ARRN; B cells (B1–B5) in the MRRN; and Mauthner (Mau) and auxiliary Mauthner (AM) cells in the MRRN. In the lamprey, the functions of Müller and Mauthner cells are unclear (reviewed in (70)). Müller cells are rhythmically active during locomotor behavior and can influence burst activity in spinal CPGs, but they are neither necessary nor sufficient for swimming behavior (70, 71), unlike smaller RS neurons (49, 61) (see Sect. 6.2.3). However, Müller cells might contribute to certain aspects of locomotion, such as rapid speed changes, fast directional maneuvers, or rapid postural adjustments (70). In lampreys, stimulation of Mauthner cells elicits weak flexure movements of the body but not the typical C-start escape responses that are initiated by these neurons in fish (reviewed in (45)).
Following spinal cord transections at 10% BL in larval lampreys, HRP retrograde labeling indicates that descending axons of Müller and Mauthner cells project for progressively greater distances caudal to a healed spinal transection site with increasing recovery times (3–32 weeks) (16). Some of these identified RS neurons can extend their axons for as much as 57 mm below a spinal lesion site (16). Large, identified RS neurons normally have long axons that are injured by rostral spinal transections and subsequently extend across the lesion site, thus satisfying the two criteria for axonal regeneration.
The lamprey brain also contains many moderately small, unidentified descending brain neurons, ∼80% of which are RS neurons (15). As stated earlier, small, unidentified RS neurons are both necessary (49) and sufficient (61) for initiating spinal locomotor activity. For these neurons, double labeling with fluorescent tracers has been used to distinguish between axonal regeneration and developmental mechanisms following SCI. The use of rhodamine dextran amine (RDA, 10 kDA; Invitrogen, Carlsbad, CA USA) and fluorescein dextran amine (FDA, 3 kDa; Invitrogen) can sometimes result in “bleed through” labeling of descending brain neurons (72), in which one tracer is visible with the optical filter for the second tracer. Obviously, “bleed through” severely compromises the accuracy of double-labeling results. Texas red dextran amine (TRDA, 10 kDa; Invitrogen) and Fluoro-Gold (FG; Fluorochrome, Englewood, CO, USA) do not display “bleed through” labeling (72), although a special application procedure is required to prevent FG from entering the circulation and labeling non-neuronal cells on the dorsal surface of the brain that obscure some of the RS neurons of interest.
< div class='tao-gold-member'>
Only gold members can continue reading. Log In or Register a > to continue
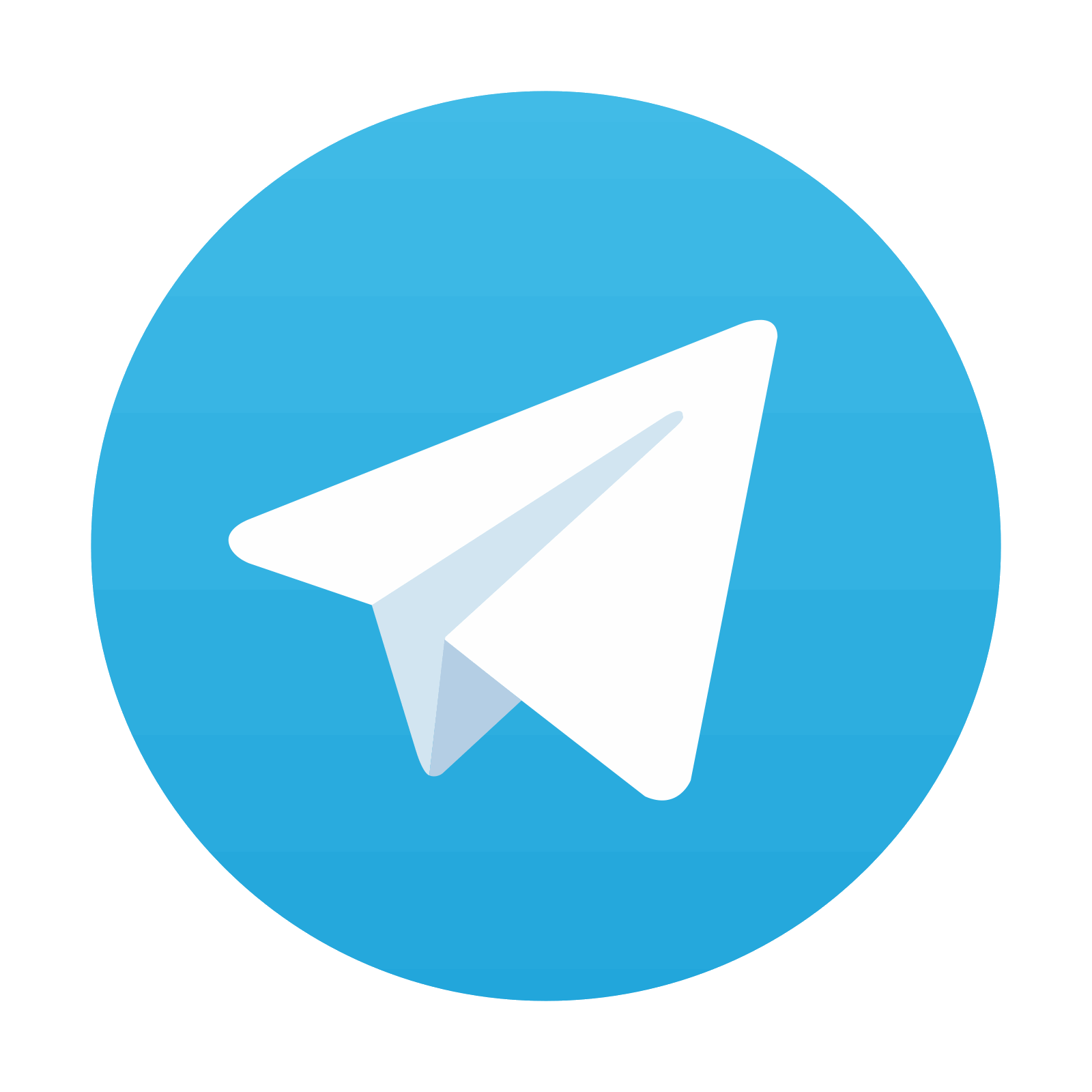
Stay updated, free articles. Join our Telegram channel
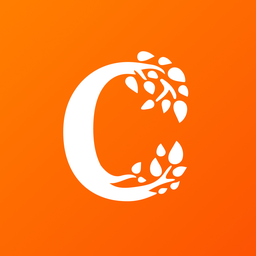
Full access? Get Clinical Tree
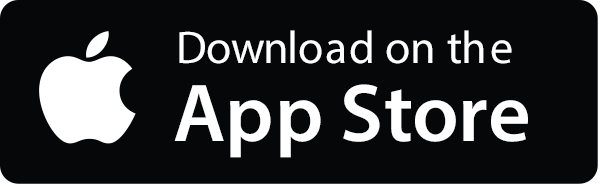
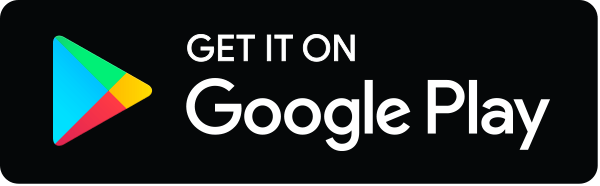