Nerve cell disability
Environmental factors
Nerve cell body response
Presence of growth inhibiting factors
Scar tissue formation
Formation of cavities and cysts at, below, and above the level of injury
4 Regeneration Obstacles
4.1 Nerve Cell Disability
Following axon injury, a shift in gene expression from a “transmitting mode” to a “growing (regenerating) mode” among damaged neurons is seen as a sign of nerve cell adaptability. Neurons with axons in the central nervous system are able to maintain a sustained expression of regeneration-associated genes (RAGs) only for a limited period. The injured neurons gradually enter an atrophic state and may, eventually, degenerate and die as a result of the absence of sufficient intrinsic and extrinsic growth stimulating factors.
4.2 Environmental Hostility
Neurons which attempt to regenerate are exposed to a growth-inhibitory environment which is created like a three-step rocket after the injury. Firstly, myelin-associated growth inhibitors such as Nogo-A, myelin-associated glycoprotein (MAG), and oligodendrocyte myelin glycoprotein (OMgp) produced by oligodendrocytes inhibit axonal growth (32). Secondly, a chemical barrier composed of chondroitin sulfate proteoglycans (CSPGs) and collagens is produced that together with components from astrocytes, microglial cells, oligodendrocytes, and meningeal cells contribute to the formation of a “scar tissue.” Finally, necrotic and apoptotic cell death in the injured area enhances the development of cavities and cysts in the spinal cord itself after many weeks. The following part of this presentation will describe obstacles of interest and address regeneration strategies to prevent those mechanisms (33–37).
5 Regeneration Strategies
Successful regeneration in the spinal cord requires the combination of several approaches divided as suggested below in order to fulfill the aims of bridging the lesion gap at the site of the SCI, replacing dead cells and to contributing to an non-hostile environment for axon regeneration:
Nerve cell strategy
Overcoming the intrinsic growth deficient neuronal capacity
Environmental strategies
Inhibiting the early inhibitory mechanisms for axon growth in the environment of the injured neurons
Counteracting the late inhibitory mechanisms on axon growth in the environment of the injured neurons
5.1 Nerve Cell Strategy
5.1.1 Overcoming the Intrinsic Growth Deficient Neuronal Capacity
The nerve cell targeted strategy aims at increasing the intrinsic neuron capacity for regeneration. Following injury, the normally inactive regeneration “program” becomes activated in order to rebuild the lost part of the axon and restore lost connections (38, 39). This program includes activation of RAGs and is significantly stimulated by treatment with specific growth factors, also referred to as neurotrophic factors (NTFs; Table 2). Their activities in general promote neuron survival and axonal outgrowth, as well as the maintenance of neuron–target interactions. In the context of SCI they stimulate survival of injured neurons, regulate the expression of RAGs in a way that increases the regeneration capacity of the injured neurons. However, as a result of this stimulation, NTFs may also cause undesired axonal growth leading to, e.g., neuropathic pain, or new functionally inappropriate, or unproductive connections. Furthermore, since NTFs act on multiple neuronal populations in the nervous system they may cause side effects, due to their action on uninjured neurons. Future research must determine which growth factors are optimal for promoting survival and regeneration of different types of neuron populations, at what time point they should be administered, and in which fashion the delivery should be performed in order to guarantee an efficient and controlled treatment. Until now, intrathecal delivery through osmotic pumps containing genetically modified neurotrophin releasing cells, e.g., fibroblasts, the implantation of slow-release cell-free systems, or gene therapy, has been possible mainly experimental options (Table 3). Unfortunately clinical trials using delivery of growth factors for various disorders have failed due to lack of efficacy or unacceptable side effects or both. However, at least one phase III study regarding efficacy and safety of growth hormone treatment in SCI is performed with the aim to study whether growth hormone can improve motor function. The growth hormone is given subcutaneously daily for 1 year in a double blinded fashion with a follow-up after 1 year (40). In the future, combining the delivery of promising growth factors with other therapeutic approaches seems to be the mode by which growth factors will be administered.
Table 2
Examples of growth factors (neurotrophic factors)
Neurotrophic factors | Abbreviation |
---|---|
Nerve growth factor | NGF |
Neurotrophin 3 and 4 | NT-3/NT-4 |
Brain-derived neurotrophic factor | BDNF |
Fibroblast growth factor | FGF |
Glia cell line derived neurotrophic factor | GDNF |
Table 3
Strategies stimulating the neuronal intrinsic regenerating capacity
Target/mechanism | Delivery | Effect |
---|---|---|
CNS-tissue (neurons) | Intrathecal injections | Regrowth of damaged axons |
Stimulating regeneration-associated genes (RAGs) | Local deposition and others | Functional improvement |
5.2 Environmental Strategies
5.2.1 Inhibiting the Early Inhibitory Mechanisms for Axon Growth in the Environment of the Injured Neurons
Strategies that obstruct the early inhibitory mechanisms on axon growth in the environment of the injured neurons involve measures that can be taken as long a formation of scar tissue is estimated to take place at the level of injury. Here, we discuss some of the main strategies that have been adopted in the early post-lesion period to counteract the accumulation of myelin-associated inhibitors and by factors that decrease the formation of scar tissue in the environment surrounding the nerve cell (34, 35, 37, 41) (Table 4).
Table 4
Strategies to counteract the early inhibitory mechanisms of the CNS environment
Factor | Target | Delivery | Effect |
---|---|---|---|
Antibodies (IN-1) | Nogo-A receptors on regenerating axons | Pumps Pills? | Blocked inhibition of axonal outgrowth Immunization |
4-Amino-pyridine | Demyelinated axons | Intravenous Intrathecal | Restored signal transmission |
Monoclonal antibody | ICAM | Immunization | Reduced edema increased spinal cord blood flow (SCBF) |
Interleukins | Systemically Intraperitoneally | Switches off the inflammatory response | |
Activated macrophages Chondroitinase ABC Erythropoietin (EPO) Rho | Proteoglycans Actin cytoskeleton | Injury epicenter Local infusion Systemic Injury site | Improved motor function Reduces cyst formation Digestion of scar Functional improvement Retain neuroprotective properties Improves SCBF and locomotion |
Blocking Axonal Growth Inhibitory Molecules
The uninjured spinal cord contains powerful inhibitory substances that prevent neurite growth. Following trauma, the same factors create a major obstacle by delaying and/or preventing the onset of the regenerating process (42, 43). The myelin growth inhibiting factor NOGO-A, a myelin protein produced by oligodendrocytes, exerts its inhibitory effect by blocking specific Nogo-A-receptors on the surface of the axons resulting in a receptor-mediated inhibition of axon growth (Table 4). Administration intrathecally of neutralizing antibodies (IN-1) that either binds to the Nogo-A itself or act as antagonists to the Nogo-A receptor blocks the effect of Nogo-A and thereby indirectly stimulates axon regeneration. Results from a Phase I trial with intrathecal administration of human anti-Nogo-A antibody in spinal cord injured patients indicate that this treatment is feasible and safe. Based on these observations, a Phase II study is underway (44).
The myelin components MAG and OMgp exert their effect in a similar receptor-mediated fashion. Anti-MAG is a monoclonal antibody directed against MAG which is a protein that inhibits axonal regeneration. The first anti-MAG administration to human has been completed (45). It is a Phase I study and the anti-MAG is given as an intravenous infusion.
Combination therapies targeting MAIS (myelin-associated inhibitors) have recently been reported and combination treatments are the future approach when using these antibodies (46).
Restoring Impulse Propagation by Modulating Axon Membrane Properties
After contusion injuries, the most frequent cause of SCI, many axons survive both primary and secondary events. However, degeneration of oligodendrocytes, mainly by apoptosis, leads to partial or total destruction of the myelin that covers the axons. As a result of this demyelination impulse propagation is severely disturbed or completely blocked. In addition, propagated electrical impulses will be spread between the demyelinated axons like a short circuit in an electrical cable when the outer insulation is damaged. An increased number of potassium channels are exposed when an axon is demyelinated and potassium (K+) leaks into the extracellular space resulting in conduction failure. Infusion of the fast voltage-sensitive potassium channel blocker Fampridine-SR (4-amino-pyridine, 4-AP) blocks the potassium channels at those gaps which results in increased action potentials, thereby facilitating propagation of action potentials in demyelinated axons (Table 4). A Phase II study published in 2007 showed an increased effect of Fampridine on spasticity when given in a tolerable dose (47). A Phase III clinical trial showed a trend for improvement only in spasticity and the therapeutic use of this drug is now focused on multiple sclerosis.
Modulating the Immune System
The inflammatory response after SCI includes degenerative and reparative processes summarized into three consecutive periods: The cellular debris and breakdown products are eliminated during the initiation period (the phase of disintegration), thereby promoting the restoration of a beneficial environment for surviving cells. This period is followed by the maintenance period (the scar forming phase) during which the trauma area is isolated from surrounding healthy tissue. Finally, the phase of shutting off macrophages, transformed from mononuclear cells in the phagocyte system, plays a key role in inflammation and an inadequate macrophage response may be an important factor behind the failure of axon regeneration in the spinal cord (48, 49). Most of the macrophages originate from microglia although the astrocytes exert a tight control of the activation of microglia to fully developed macrophages, thereby preventing rapid removal of, e.g., growth inhibitors that are present in degenerating myelin. Thus, an optimal phagocytic response may benefit from a facilitated entry of monocytes into the degenerating spinal cord white matter and their subsequent differentiation to competent macrophages, provided that this will not result in a fully developed inflammation. Recruitment into the spinal cord of circulating monocytes requires that they first attach to the endothelial cells in capillaries before entering the spinal cord tissue. This attachment and the subsequent entry are regulated by cell adhesion molecules (CAMs) located at the cell surface (50).
Intercellular adhesion molecule (ICAM) at the surface of endothelial cells is a ligand for macrophages and plays a key role in the initiation phase of the inflammatory response (Table 4). It might be possible to regulate the monocyte-mediated components of inflammation following SCI by modulating ICAM expression. A sufficient level of macrophage activity in the phase of initiation, followed by a downregulation of this activity in the phase of maintenance, would first promote rapid removal of cellular debris and reduce the impact of macrophage in the scar forming phase, respectively.
The interleukin (IL) family of cytokines is important modulators of immune and inflammatory responses following neural trauma. Whereas IL-1 and IL-6 are considered proinflammatory, IL-10 plays a role in switching off the inflammatory response (Table 4). Intravenous or intraperitoneal administration of IL-10 attenuates the inflammatory response and mitigates the secondary injury process. Interleukins have been shown to reduce edema formation, reduce the period of secondary damage, and improve local blood flow and neurological recovery following trauma to the spinal cord. In conclusion, ICAM and interleukins are molecules potentially useful for pharmacological treatment in SCI (51).
Activated Autologous Macrophages
Macrophages clean up cell debris promoting a controlled inflammatory reaction that forms the first phase of the wound healing process. This does not occur in CNS because their activities are prevented by a mechanism known as immune privilege. In addition, CNS macrophages are significantly less activated compared to macrophages in the peripheral nervous system following injury. This may also contribute to less efficient removal of myelin-associated inhibitors and suboptimal production and release of growth factors. However, it appears that incubated microphages circumvent the immune privilege and thereby support regrowth of axons through the injury site, enabling some recovery of neurological function (52, 53). Implantation of activated macrophages derived from peripheral nerves has been shown experimentally to have neuroprotective effects and stimulate axonal regeneration (Table 4). These findings were the basis for the initiation of a Phase I clinical trial in patients with SCI, using activated macrophages isolated from the patient’s own blood (ProCord). The following phase II study has however been suspended (54) and no clinical study is ongoing.
Chondroitinase ABC: A Molecular Machete
Proteoglycans, collagens, and adhesive proteins in the extracellular matrix participate in the scar formation which is hostile towards axonal regeneration but a natural response after injury (55). Chondroitin sulfate proteoglycans (CSPGs) are the main contributors to the scar formation and the major culprit of the failure of injured axons to grow beyond the level of injury. A bacterial enzyme was discovered in 2002 with the capability to digest chondroitin sulfate (56). Bacterial chondroitinase ABC (ChABC) given intrathecally following a trauma in the posterior horn in adult rats reduced scar formation by acting as a molecular machete (Table 4). As a result, regeneration of sensory and corticospinal axons was supported, the level of regeneration-associated proteins was increased, and functional recovery of movements and proprioception was observed. The use of implanted channels containing Schwann cells together with ChABC and olfactory ensheathing cells (OECs), as an example of combined treatment, promoted regeneration of serotonergic axons beyond the grafts (57, 58). Finally, in a clinical Phase III setup, ChABC has been tested following herniated lumbar discs resulting in less leg pain in the treatment group compared to controls (59).
Erythropoietin
Erythropoietin (EPO), the prime stimulator of erythroid progenitor cell proliferation, has been shown to exert neuroprotective effects by reducing lipid peroxidation and inflammation as well as by counteracting apoptosis and stimulating oligodendrogenesis (60) (Table 4). Administration of carbamyl erythropoietin (CEPO) within the first 24 h following experimental SCI reduces the neurological deficits compared to controls (61). However, two clinical trials using EPO following spinal shock and spinal cord compression have been suspended and no further clinical studies along this line are ongoing.
C-AMP and RHO Pathway Antagonists
Altering the intracellular signaling events following inhibition of neurons has been investigated as a therapeutic strategy for SCI. By increasing the intracellular levels of cyclic adenosine monophosphate (c-AMP) and protein kinase A activity, neurons were shown to overcome myelin-based inhibition. Cyclic AMP is able to turn on growth factor genes in nerve cells, thereby stimulating axon growth and helping to overcome signals that normally inhibit regeneration. Post-injury administration to zebrafish of Rolipram, an agent that prevents the hydrolysis of c-AMP and thereby increases c-AMP levels, lead to regeneration of severed CNS axons and restored function. Increasing c-AMP levels after SCI has also been tried in combination with other treatments such as Rolipram and Schwann cell transplants resulting in improved function in paralyzed rats (62). Before any human clinical test can begin, the therapeutic windows of c-AMP delivery must be defined as well as the role of the substance in various combination treatments.
The GTPase-associated signaling protein Rho influences cell motility by transducing extracellular signals to alterations in the actin cytoskeleton (63) (Table 14.4). SCI increases Rho-activity resulting in growth cone collapse and neurite retraction. Promoted neurite extension, improved spinal cord blood flow, and improved locomotion have been seen after administration of Rho-associated kinase inhibitors early after experimental SCI (64). A cell permeable variant of Rho inhibitor known as Cethrin has been developed and used in a Phase I/IIa study (65). Cethrin is an engineered version of C3 transferase derived from clostridium botulinum. The drug blocks the common pathway, called RHO, through which myelin-related inhibitory proteins affect the spinal cord nerve cells. Cethrin was administered epidurally within 72 h after SCI simultaneously with decompression surgery. The drug seems to be safe and individuals with cervical injuries improved better than expected both in sensory and motor scores. Hopefully, a future prospective randomized clinical study will bring more knowledge about the therapeutic effect of this drug.
5.2.2 Counteracting the Late Inhibitory Mechanisms on Axon Growth in the Environment of the Injured Neurons
This period starts once the scar formation is established. Morphologically the upper and lower stumps of the injured area are separated by a gap consisting of intra- and extramedullary scar tissue and/or cyst formations, which creates a mechanical barrier and prevents injured axons to navigate this area. The growing axons usually fail to re-enter the spinal cord above or below the lesion and they need to be guided through or around the mechanical obstacle by biological or biosynthetic bridges or supportive structures with or without the combination of growth factors. The term “filling the gap” includes a variety of bridging strategies to overcome this mechanical obstacle and the combination of supportive bridging structures and specific cell implantations may be a promising way to support and maintain axon elongation across the injury site. Cellular transplantation after SCI has in that respect several aims: to bridge “the gap,” to replace dead cells, and to contribute to an affirmative environment for axon regeneration and transplants and can be more or less used as a complement to the following supportive structures.
Supportive Structures
Supportive structures promote axonal growth by acting as a scaffold for growth factors and/or as a substrate for growth interactions with regeneration axons. Peripheral nerve tissue or its components are the most frequently used scaffold due to the ability of injured axons to grow long distances in a transplanted peripheral nerve graft.
Peripheral Nerves
Peripheral nerve grafts with different combinations of therapies have been utilized. An elaborate example of the use of peripheral nerve grafts to bridge SCI is the studies by Olson and collaborators (66) (Table 5). In their initial experiments, multiple intercostal nerve grafts were inserted between the stumps of a complete spinal cord transection to provide conduits across the injury site from gray matter caudally to white matter rostrally for ascending pathways and from white to gray matter for descending pathways. The nerve grafts were stabilized with fibrin glue allowing slow release of acidic fibroblast growth factor (aFGF). Morphologically, axonal regrowth across the lesion site was seen as well as recovery of hindlimb sensorimotor function. Similar results were subsequently obtained even after a chronic lesion (67). Furthermore, clear evidence for at least a partial causal connection was established in a term experiment in which functional recovery was completely reversed after sectioning the grafts (67). However, much work remains to be done to determine whether therapies that involve peripheral nerve bridge grafting can safely and effectively improve outcome after human SCI.
Table 5
Strategies to overcome the late inhibitory mechanisms of the CNS environment: supportive structures
Factor | Target | Delivery | Effect |
---|---|---|---|
Peripheral nerve + aFGF | Axotomized axons | Locally with glue | Structural and functional recovery |
Schwann cells (SC) + growth factors | Axotomized axons | Placement of SC transplants in guidance channels | Reduced secondary degeneration Functional improvement |
Olfactory ensheathing cells (OEC) | Axotomized axons | Local deposition | Reduced secondary degeneration Functional improvement |
Non-cellular elements + stem cells | Cut axons Scar forming glia | Placement of stem cells in transplanted polymers | Improved tissue sparing Reduced scar formation Functional improvement |
Schwann Cells
Schwann cells are the essential growth promoting cellular element of peripheral nerves. Isolated Schwann cells or growth promoting Schwann cell molecules would therefore be suitable as guidance channel for injured spinal cord axons. This approach was used by Bunge and co-workers who filled “guidance channels” with Schwann cells and growth factors (68) (Table 5). Increased axonal growth reduced secondary degeneration of axons and functional recovery was observed in the animal models (69). The hostile astrocytic reaction towards Schwann cells hampers, however, the ability of these cells to produce myelin. The first remaining part towards human clinical trials is to conduct safety and efficiency evaluations of transplanting autologous Schwann cells into non-human primates after contusive SCI. There are no reports of ongoing clinical trials involving transplantation of Schwann cells after SCI.
Olfactory Ensheathing Cells
Olfactory ensheathing cells (OECs) associate with olfactory sensory axons along their way to the target neurons in the olfactory bulb. OECs are continuously supporting the growth of axons into the olfactory bulb from newly formed olfactory sensory neurons in the olfactory mucosa. OECs are fully integrated into the adult CNS; they release growth factors and other growth promoting molecules and are able to produce myelin around demyelinated or regenerating CNS axons. OECs stimulate regrowth of damaged axons in the spinal cord when locally implanted as well as growth of axons through areas with scar-tissue formation resulting in signs of functional recovery of sensory and postural functions (Table 5). In addition to their supportive and guiding properties, OECs have the ability to promote synaptic plasticity and to contribute to structural and functional repair when stem cells from olfactory mucosa are present in the transplants. The hostile astrocytic reactions which are seen with Schwann cell implants are essentially absent after implants of OECs making those cells more favorable candidates than Schwann cells for supporting axonal regeneration in injured CNS issue. OECs for transplanting purposes can be harvested under local anesthesia from human olfactory mucosa, grown in vitro and thereafter used for transplantation.
Among other studies a Phase I/IIa design study whose main aim was to test the feasibility and safety of transplantation of autologous olfactory ensheathing cells into the injured spinal cord in human paraplegia was published in 2008 (70). The authors concluded that transplantation of autologous olfactory ensheathing cells into the injured spinal cord is feasible and safe up to 3 years of post-implantation. However, this conclusion should be considered preliminary because of the small number of trial patients. For an extended review see (71). In summary, issues like optimal source of cells, their age, and also graft strategy are not yet fully answered but studies in humans have in any case not shown any adverse side effects.
Artificial Supportive Structures
A variety of artificial biocompatible materials have been used to bridge SCI (72). As an example Rochkind and colleagues placed a multi-component polymer filled with stem cells coated with a suitable substrate for axonal growth into the gap of the hemisected spinal cord (73). Improved hindlimb functional recovery was seen in the grafted animals compared to the controls (Table 5).
Transplantation of Embryonic/Fetal Neural Tissue for Spinal Cord Repair
The use of embryonic/fetal neural tissue for repair of CNS disorders including SCI has played an important role in establishing the principle that immature neural tissue/cells are able to survive, differentiate, and promote functional recovery in experimental animal studies. Embryonic/fetal neural tissue has a number of properties, which makes it attractive in SCI, although ethical aspects complicate the use of this tissue both for experimental and clinical purposes (Table 6). The tissue must have the potential to survive transplantation into the mature and injured host spinal cord and further to thereafter differentiate to mature neurons with the ability to make functionally useful synaptic connections. Embryonic/fetal neural tissue also contains stem cells and non-neural cells with trophic and substrate supporting ability for transplanted immature neurons as well as for damaged neurons in the host spinal cord (Table 6).
Table 6
Properties of embryonic tissue
1 | Rapid growth and cell division |
2 | Ability to develop to similar cells as in surrounding tissue |
3 | Less often rejected |
4 | Contain factors stimulating in growth of vessels as well as a higher proportion of growth factors promoting survival when transplanted |
5 | Less sensitive to ischemia and thus able to survive in surroundings with lowered oxygen levels |
The ideal cellular candidate for cell-based repair strategy should therefore be able to:
Support axonal elongation and regrowth through the adult CNS.
Restore myelin around spared axons.
Migrate within the CNS to appropriate place(s) and coexist with astrocytes.
Fully integrate within the lesion microenvironment.
Different strategies have been used in transplanting embryonic/fetal neural tissue in experimental spinal cord injuries (Table 7) and the transplantation procedure has five primary objectives:
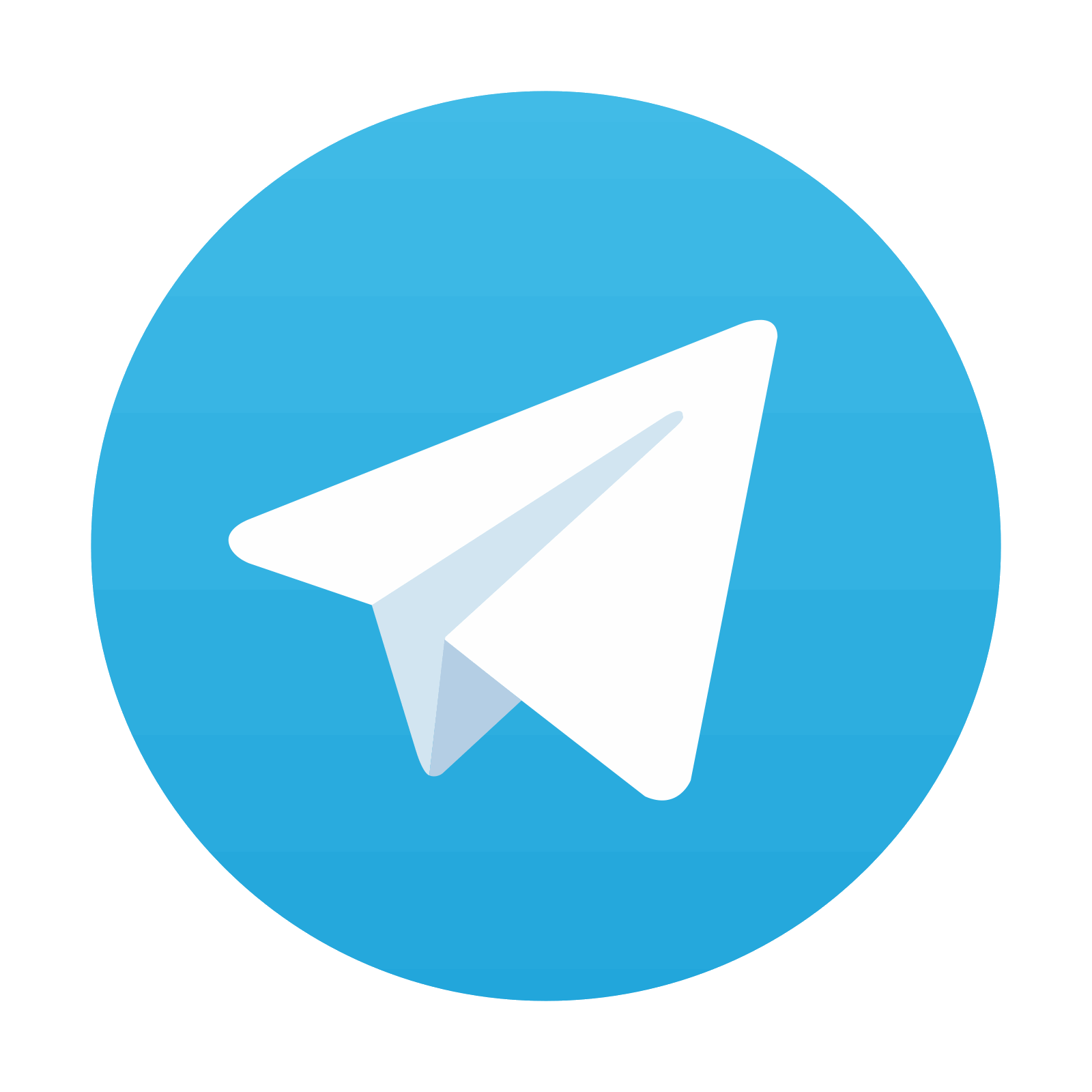
Table 7
Strategies to overcome the late inhibitory mechanisms of the CNS environment: transplantation of embryonic/fetal tissue
Factor | Target | Delivery | Effect |
---|---|---|---|
Solid embryonic/fetal tissue | Red nucleus axons | Transplantation to cavity | Rescue axotomized neurons |
Brain stem fragments | Denervated terminal field | Injection | Local reinnervation Functional restitution |
Solid embryonic/fetal tissue | Axotomized axons | Transplantation to cavity | Axonal elongation into and out of transplant |
Human embryonic/fetal tissue | Axotomized axons | Transplantation | Feasibility Cyst obliteration |
To replace specific cells in order to restore lost functions.
To minimize neuron degeneration and scar formation.< div class='tao-gold-member'>Only gold members can continue reading. Log In or Register a > to continue
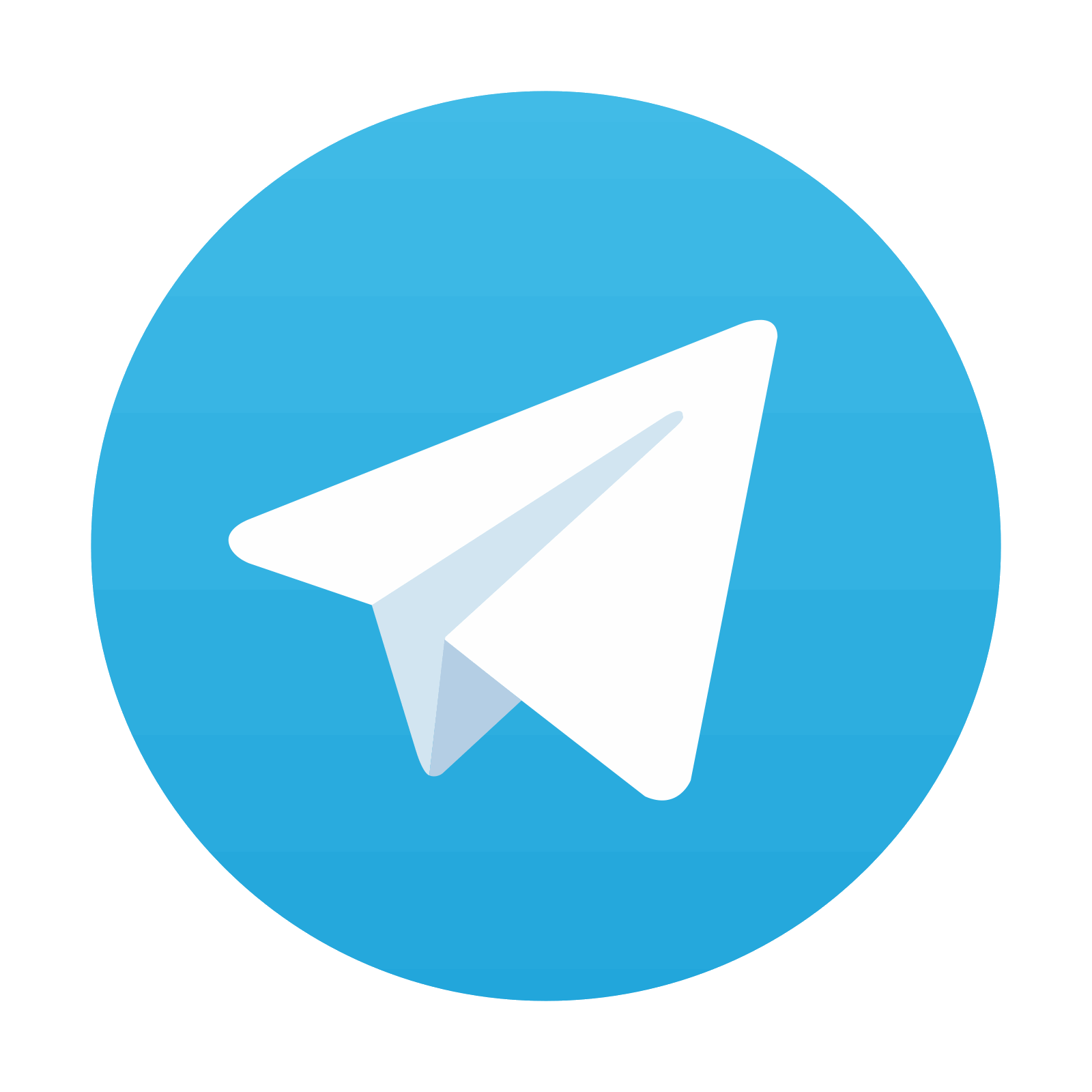
Stay updated, free articles. Join our Telegram channel
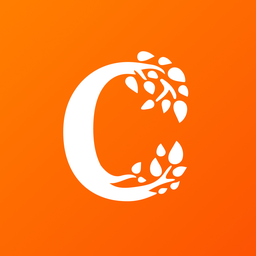
Full access? Get Clinical Tree
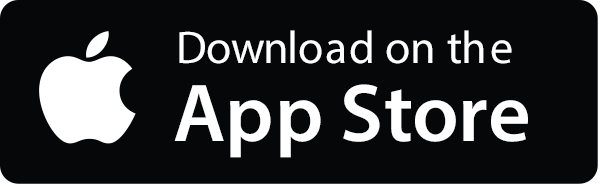
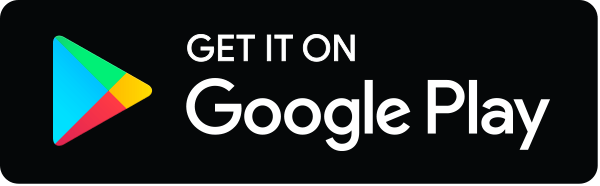
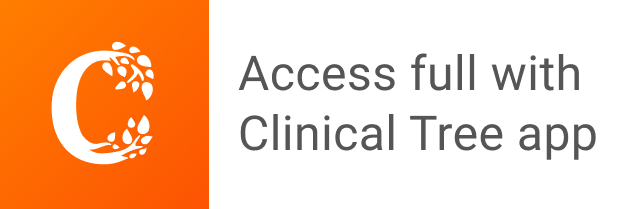