4 Sperm Preparation for Fertilization Bart M. Gadella* Fertilization is a decisive moment in life and enables the combination of the DNA from two gametes to ultimately form a new organism. The sperm surface, especially the head area, has distinguishable functional areas that are involved in distinct fertilization processes. It is known that the sperm surface undergoes constant remodelling during its migration, especially in the epididymis (maturation) and in the oviduct (capacitation). Altogether, these changes serve to prepare sperm to meet the oocyte and bind to the zona pellucida. To this end, not only must sperm acquire hyperactivated motility but also three independent, successive events are required to establish monospermic fertilization. Firstly, the sperm cell must secrete its acrosome contents (the acrosome reaction), thus allowing the sperm to penetrate the zona pellucida and to reach the oocyte plasma membrane – the site of fertilization. Next, the sperm cell binds to the oocyte plasma membrane, and the two different cells fuse together. Finally, the just fertilized oocyte must protect itself from polyspermic fertilization, which is established by the secretion of the cortical granule contents over the entire oocyte cell surface into the perivitelline space (the cortical reaction). Altogether, these events lead to the activation of the oocyte and the formation of one male and one female haploid pronucleus. After syngamy, the resulting diploid zygote is ready to develop into an embryo and a new organism. This chapter provides an overview of the current understanding of the preparative processes of sperm that are required to achieve mammalian fertilization. In addition, the consequences of semen processing, e.g. for semen storage or for flow cytometry sorting, for sperm integrity and its fertilization capacity are discussed. In the last moments of spermatogenesis, sperm cells are released from the Sertoli cells and from the syncytia of synchronously formed spermatids into the lumen of the seminiferous tubules. The released sperm cells are starting a complex and lengthy process, which should bring them eventually to the cumulus oocyte complexes in the oviducts of the female. During the transit through the male and female reproductive tracts, alterations of spermatozoal metabolism, motility and surface properties occur that allow the sperm to properly interact with the extracellular vestments of the oocyte and to eventually bind to and fuse with the oocyte. After fertilization, the sperm cell also provides a trigger to the oocyte to prevent polyspermic fertilization. Some of the ergonomics of a typical mammalian sperm cell are already apparent at the moment they are released in the testis. Typically, spermatozoa consist of a head, a midpiece and a tail (for details on sperm structure, see Eddy and O’Brien, 1994) Within the sperm head, two large intracellular compartments can be distinguished: the acrosome and the nucleus. The latter contains the male haploid genome, which is highly condensed, with protamines having replaced histones almost completely during spermatogenesis (for a review see Dadoune, 2003). This results in the chromatin being approximately 10× more compact than somatic cell chromatin, so the proportions of the sperm head are minimized. Moreover, as a haploid cell, the sperm contains only half the complement of DNA of somatic cells. However, the dimensions of the nucleus as seen under a microscope can be misleading: the somatic nucleus is round and ball shaped, while the sperm nucleus of farm animals and equines is extremely flattened (oval and pancake shaped). Furthermore, the extremely compacted DNA is less vulnerable to enzymes (apoptotic related), toxins or oxidantmediated damage. The nucleus is then ‘protected’ and compact, both of which are requirements for the optimal transport of the spermatozoa. The acrosome is located at the apical edge of the sperm head, while the flagellum develops at the distal part of the sperm head. The acrosome is a cap-shaped organelle with an acidic pH and is filled with hydrolytic, glycosidic and proteolytic enzymes (Moreno and Alvarado, 2006). These enzymes are condensed into the so-called acrosomal matrix in the lumen of this organelle. The contents of the acrosome are enclosed by one continuous membrane, although the part directly overlying the nuclear envelope is referred to as the inner acrosomal membrane, while the part associated with the sperm plasma membrane is referred to as the outer acrosomal membrane. After the acrosome reaction, the contents of the acrosome are involved in sperm binding to the zona pellucida of the oocyte and its penetration (Gadella and Evans, 2011), and possibly sperm–cumulus association as well (Sun et al., 2011); the acrosome contents may also contain factors involved in sperm–oocyte binding and fusion (Sachdev et al., 2012). The sperm midpiece is connected by a connective element to the sperm head and contains a battery of less than 100 mitochondria that are coiled around the flagellum and elongate further down the tail (Bahr and Engler, 1970). It is in the mitochondria of the midpiece that aerobic metabolism occurs (the head and tail are devoid of mitochondria). The ATP produced probably serves to provide the energy for the motility machinery and for housekeeping processes through the sperm membrane. The constantly changing environments of the spermatozoa discussed later in this chapter probably result in adaptive ionic and metabolic processes that are important for maintenance of the intracellular milieu (Shivaji et al., 2009; Ramió-Lluch et al., 2011). The tail is the mitochondria-free distal part of the flagellum. This part of the flagellum allows sperm movement. The microtubules of the sperm tail effectively slide over each other, resulting in bending of the tail. Microtubule sliding is an ATP-consuming process, fed by ATP produced either in the midpiece (aerobically) and/or in the tail itself by glycolysis. The central flagellum is surrounded by a fibrous sheath, in which there is high-capacity glycolytic machinery. This sheath provides the tail elastic properties, which are required for the forward-propelling properties of the sperm tail (for a review see Eddy, 2007). The sperm head, midpiece and tail have their own specializations and ergonomic adaptations that allow the cell to optimally reach and fertilize the oocyte. One aspect of this is that sperm cells have lost various somatic cell processes and organelles. For example, during spermiation, the sperm cell loses its capacity for transcription and translation (Boerke et al., 2007). Once the remaining and specialized organelles are organized as mentioned above, sperm cells also completely lose membrane transport capabilities via vesicles, e.g. they have no endocytosis, exocytosis or inter-organelle transport. A number of other ‘typical’ cellular components are also absent, including ribosomes (no translation), Golgi complexes, endoplasmic reticulum, peroxisomes and lipid droplets. Sperm cells only contain a minimal amount of cytosol as well (for a review see Eddy and O’Brien 1994). All these features are in fact signs of the minimalization of sperm volume and size, and provide evidence that the cell is terminally differentiated and prepared for only one last task, namely the fertilization of the oocyte. After spermiation, the sperm cell in the testis is not competent to fertilize the oocyte, but it already shares all of these ergonomic features with the activated sperm in the oviduct, which has at that point become competent to fertilize the oocyte. In the testis, the sperm cell is not motile, nor does it have the appropriate surface organization or proteins to recognize the cumulus–oocyte complex (Boerke et al., 2008). The sperm cell must undergo a series of posttranslational and environmental modifications that will result in its ability to undergo the fertilization tasks. The current understanding of the post-testicular sperm remodelling will be reviewed here, and suggestions made for future research efforts to fill the gaps in our current knowledge. This section focuses on the domained structure of the sperm surface and discusses its importance for fertilization. Functionally, the sperm head surface can be divided into three regions: apical, equatorial and post-acrosomal. The apical area of the sperm head is involved in sperm–zona recognition (primary zona binding) and consequently exclusively contains the primary zona-binding proteins that are recruited to this apical surface area only shortly before binding takes place (in the oviduct or in in vitro fertilization (IVF) media) (van Gestel et al., 2005, 2007). The series of events that are induced after sperm–zona binding are depicted in Plate 3. After the sperm has bound to the zona pellucida, the acrosome reaction occurs and results in the exposure of the acrosomal enzymatic matrix to the zona pellucida (the secondary zona-binding proteins). At this stage, zona binding also results in zona digestion. To ensure maximal exposure of the enzymatic matrix to the zona pellucida, simultaneous multi-point membrane fusion occurs between the outer acrosomal membrane and the plasma membrane. This causes the formation and release from the sperm cell proper of hybrid vesicles of these two membranes. The resulting hybrid vesicles are sometimes referred to as the ‘acrosomal shroud’. Only two-thirds of the apical side of the outer acrosomal membrane is involved in this very characteristic fusion process (Tsai et al., 2012). It is of note that it has recently been established that boar sperm capacitation involves the docking of the sperm acrosome to the sperm surface (Tsai et al., 2010) and that the initiation of the acrosome reaction may be earlier than at zona binding, at least in the mouse (Jin et al., 2011; Sun et al., 2011). The remaining unfused part of the sperm surface and the outer acrosomal membrane forms the so-called ‘equatorial segment’. In this area, the plasma membrane has become continuous with the one-third section of the outer acrosomal membrane, which was already continuous with the inner acrosomal membrane (Tsai et al., 2010). This continuous membrane structure takes over the surface function of the released apical plasma membrane, thereby ensuring the maintenance of an intact intracellular milieu. The resulting equatorial segment has recruited a specific machinery of proteins that will be involved in sperm–oolemma binding and fertilization fusion. In part, these proteins originate from the acrosomal membrane (Ito et al., 2010; for a review see also Gadella and Evans, 2011). Thus, the sperm head surface contains at least three functional domains involved in gamete interaction: one involved in zona recognition (apical area), one in the acrosomal fusions (the apical and pre-equatorial area) and one in the fertilization fusion (the equatorial segment). The function of the postacrosomal region of the sperm plasma membrane during the process of fertilization is not known. In the testicular sperm cell, many of the components required for fertilization are not present and need to be recruited from the post-testicular environments (van Gestel et al., 2007; Leahy and Gadella, 2011; Caballero et al., 2012). Once attached to the sperm surface, these components also need to concentrate at their specific surface areas in order to give each domain its specific tasks (van Gestel et al., 2005; Zitranski et al., 2010; Watanabe and Kondoh, 2011). Also, the midpiece and tail have a dynamic domained structure, and probably modifications thereof, that are related to sperm motility characteristics. Testicular sperm cells are immotile, whereas ejaculated sperm have gained straight-forward movement, following their maturation in the epididymes. The sperm will obtain full hyperactivated motility characteristics after capacitation in the oviduct (Suarez and Pacey, 2006; Suarez, 2008). These dynamic, changing sperm features are accomplished gradually through the influence of the constantly changing extracellular environment encountered en route to fertilization. In the next section, these processes will be followed at the level of the sperm surface. Of considerable cell biological and membrane biochemical interest is the question as to how the domained structure of the sperm surface and its dynamic reordering (Gadella et al., 1995) are accomplished. A number of possible dynamic alterations in the sperm plasma membrane and interacting components of the cytoskeleton and the extracellular matrix may be involved (Fig. 4.1). The actual membrane phenomena underlying plasma membrane domain dynamics at the sperm surface are of interest and may add to the understanding of how and why certain treatments in sperm handling, storage and IVF are good or bad. A hypothetical scheme of possible interactions between the sperm surface and the components of the male or female genital tract is depicted in Fig. 4.2, and the five relevant processes shown in this figure are described below. From the various epithelia of the male and female genital tract, blebbing cytosol-filled vesicles are released into the genital fluids and then interact and exchange surface components with sperm (see process 1 in Fig. 4.2). It is unlikely that these vesicles fuse with the sperm, as this would dramatically increase sperm volume (which has already been reduced maximally in order to obtain an ergonomically designed cell optimally suited for fertilization). Blebbing of vesicles has been demonstrated in the epididymal duct and in the epithelia of the vesicular gland and the prostate of the boar (Tsai and Gadella, 2009). The function of the blebbed vesicles is largely unknown, although hypothetically they may exchange components with the sperm surface and/or modulate the repressive activity of white blood cells. Serum components are released into the genital fluids by transcytosis (Cooper et al., 1988; see process 2a in Fig. 4.2). Lipoprotein particles may invade the surroundings of the sperm and facilitate exchange at the sperm surface. Fluid-phase secretion and adsorption alter the extracellular matrix (ECM) of sperm by a variety of sticking molecules and enzymes that cause posttranslational modifications of ECM molecules (process 2b in Fig. 4.2). Apocrine secretion of exosomes may be involved in altering the sperm surface and function as well. Exosomes are secreted by the epididymis (epididymosomes), by the prostate (prostasomes) and by the uterus (uterosomes) (Gatti et al., 2005; Griffiths et al., 2008; Thimon et al., 2008) (process 3 in Fig. 4.2). Further, exosomes may provide sperm with tetraspanins, a group of membrane proteins involved in the tethering of proteins into protein complexes. The addition of CD9 (an exosome marker) originating from the oocytes on to the sperm surface by membrane particles occurs even when sperm reach the perivitelline space (Barraud-Lange et al., 2007a,b), although the function of exosomes in fertilization fusion is unclear (Miyado et al., 2008; Barraud-Lange et al., 2012). A change in the sperm’s surface properties may alternatively be elicited by sperm interactions with ciliated epithelial cells in the epididymis, uterus and oviduct (Zhang and Martin-Deleon, 2003; Gatti et al., 2004; Sostaric et al., 2008) (process 4 in Fig. 4.2). The interaction at the level of the epididymal epithelia guides the sperm through a maturation process during which a continuous modification of the sperm surface takes place, and by which the sperm cells obtain their motility characteristics and the removal of their redundant cytoplasmic droplets. The interaction with the oviduct has a physiological role in in situ capacitation, but this is not understood at the molecular level (Chang and Suarez, 2012). The importance of sperm interactions with other ciliated epithelial cells of the female and male genital tract is also not fully understood, but could be crucial for sperm surface remodelling and sperm function. When sperm enter the uterus they, together with seminal plasma components, evoke an immunological response (process 5 in Fig. 4.2) (Schuberth et al., 2008). For instance, leucocytes will infiltrate into the uterine fluid (Taylor et al., 2008) and affect the surface of sperm. This probably serves to cleanse the uterus of degenerated sperm and to prevent an inflammatory response in it (Woelders and Matthijs, 2001). The sperm involved in fertilization colonize the lower parts of the oviduct, where no leucocyte infiltration takes place. As discussed above, the domained surface of sperm is already apparent in spermatids in the seminiferous tubules of the testis before spermiation (Eddy and O’Brien, 1994). The molecular dynamics involved in the establishment of surface specialization upon spermatogenesis is largely unknown. Generally, it is clear that the polar organization of the extracellular matrix components of the cytoskeleton and the sperm cell organelles are involved in its domained and heterogeneous surface (Plate 3). As already noted, in mature sperm cells, the amount of cytosol is minimal and, indeed, the observed surface domains mirror the organelle organization located just under the sperm surface: at the apical area, the acrosome, the post-acrosomal area, the nuclear envelope, the midpiece surface covering the mitochondria and, in the tail, at the surface covering the fibrous sheath. Moreover, once liberated in the lumen of the seminiferous tubules, the sperm cells will start their travel through the male and female genital tracts and will meet a sequence of different environments. During this voyage, surface remodelling takes place, most likely at many sites in the two genital tracts: (i) upon somatic maturation in the epididymis (Gatti et al., 2004; Dacheux et al., 2005); and (ii) by recoating and decoating events induced by the accessory fluids added at ejaculation, which probably stabilize the sperm for their further journey in the female genital tract (Gwathmey et al., 2006; Girouard et al., 2008). These changing environments may cause sperm surface remodelling and thus may influence their potential to fertilize the oocyte. After release from the Sertoli cells into the lumen of seminiferous tubules, sperm cells are equipped with a number of proteins that are reported to be involved in zona binding. At its surface, the sperm has transmembrane proteins belonging to the ADAMs family. These were initially thought to be involved in the fertilization process, but are now reported to be involved in sperm–zona binding. ADAM-2, also known as fertilin b, has such a function in boar sperm (van Gestel et al., 2007). Other testicular sperm proteins, e.g. sperm lysosomal-like protein (SLLP1; Herrero et al., 2005), and sperm acrosomal membrane proteins (SAMP14 and 32; Vjugina and Evans, 2008) and Sp56 (Buffone et al., 2008), are involved in secondary zona binding, as they are localized in the acrosome and only become exposed to the zona pellucida after the induction of the acrosome reaction. Some secretory proteins, e.g. CRISP (cysteine-rich secretory proteins), are also involved in sperm–zona adhesion, sperm–oolemma binding or fertilization fusion (Busso et al., 2007a; Cohen et al., 2007; Da Ros et al., 2007). CRISP2 is from testicular origin, while CRISP1 and CRISP4 originate from the epididymis (Busso et al., 2007b). The exact mechanism of CRISP’s association with the sperm surface is not yet known, although CRISP1 is one of the abundant proteins in epididymosomes (Thimon et al., 2008). Epididymosomes are also reported to influence the lipid composition of the sperm surface (Rejraji et al., 2006). Other proteins that have been shown to be added to the sperm surface in the epididymis are SED1 (or P47). These proteins are known to have a role in oviduct and zona binding (Shur et al., 2006; Nixon et al., 2011). Angiotensin converting enzyme (ACE), which becomes glycosylphosphatidylinositol (GPI) – anchored to the sperm surface upon epidiymal maturation, is involved in the proper orienting of ADAM proteins (Yamaguchi et al., 2006; Nixon et al., 2011). During sperm capacitation, phospholipases and ACE serve to remove a proportion of GPI-anchored proteins and are believed to play a role in the remodelling of lipid rafts (Kondoh et al., 2005; Inoue et al., 2010; Watanabe and Kondoh, 2011). The addition of GPI-anchored proteins has been reported to occur in the epididymis where the sperm adhesion molecule SPAM-1 is secreted by the epithelial cells into the luminal fluid and later associates with the sperm surface (Zhang and Martin-Deleon, 2003). Likewise, in the pig, the spermadhesin AQN-3 and carbonyl reductase are added to the sperm surface (van Gestel et al., 2007). Inhibition of hamster carbonyl reductase activity results in a decreased affinity for the zona pellucida, while the sperm remain motile and intact (Montfort et al., 2002). Even under very stringent detergent conditions, AQN-3 is still able to bind to the zona pellucida (van Gestel et al., 2007). Data derived by proteomics has been useful for the identification of proteins that do not have a direct function in sperm–zona binding but are associated with the zona binding protein complex formed at the sperm surface (van Gestel et al., 2007; Gadella, 2008). Some of these proteins (such as protein phosphatases) are involved in sperm signalling processes, while others are involved in the maintenance of the redox balance (peroxiredoxin 5) (see Gadella et al., 2008). The latter include a potassium channel, which might induce membrane hyperpolarization by K+ efflux. This hyperpolarization may, in turn, open voltage-dependent Ca2+ channels that enable Ca2+-dependent processes in the capacitating sperm (Tsai and Gadella, 2009). An interesting observation has been that the major zona binding proteins listed above tend to aggregate in capacitating sperm (under IVF conditions) at the surface area that is involved in zona binding (van Gestel et al., 2005, 2007), and this coincides with the attraction of soluble N-ethylmaleimide-sensitive factor attachment protein receptors (SNAREs) involved in the acrosome reaction (Tsai et al., 2007, 2010, 2012). The fact that both outer acrosomal SNAREs and plasma membrane SNAREs are concentrated at the apical ridge area of the sperm head after capacitation has led to the assumption that the lipid-ordered membrane aggregation is a preparative step for the acrosome reaction (as proposed in Fig. 4.3).
Utrecht University, Utrecht, the Netherlands
Introduction
Sperm Ergonomics
The sperm head
The sperm midpiece
The sperm tail
Other ergonomic aspects of sperm
The Sperm Surface
General Mechanisms of Sperm Surface Modification by the Extracellular Environment
Sperm Surface Modification in the Male Genital Tract
Stay updated, free articles. Join our Telegram channel
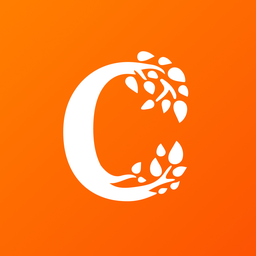
Full access? Get Clinical Tree
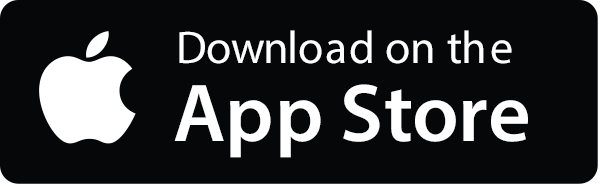
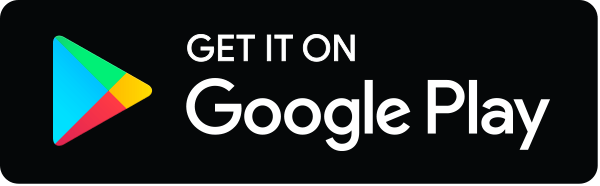