Institution/consortium
Country
Cell bank established
Information
North America
Audubon Nature Institute
USA
INA
>15 feline cell linesa
American Museum of Natural History
USA
2001
>28,000 tissue and DNA samplesa, b, c, d, e
San Diego Zoo Global Wildlife Conservancy
USA
1975
>8,000 cell lines from 800 speciesa, b, c, d
Smithsonian Institution
USA
1970
>1,000,000 tissue and DNA samples and cell lines from >18,000 speciesa, b, c, d, e
Toronto Zoo
Canada
2007
>112 cell linesa, b, c
Europe
Institute of Biomedical and Environmental Science and Technology
UK
2008
19 speciesb
Mediterranean Marine Mammals Tissue Bank
Italy
2002
>11 Mediterranean speciesa
Museo Nacional de Ciencias Naturalesf
Spain
INA
>250 individual samples of Iberian lynxa
Asia
Kunming Wild Animal Cell Bank
China
1986
1,455 cell lines from 289 speciesa, b, c, d, e
Conservation Genome Resource Bank for Korean Wildlife
Korea
2002
13,475 tissue and DNA samples from 407 speciesa, b, d
National Center for Cell Sciences
India
INA
INA
South America
Zoologico de Buenos Airesf
Argentina
2005
INA
Australia
Animal Gene Storage and Resource Centre of Australia
Australia
1995
INA
Africa
Wildlife Biological Resource Centre
South Africa
2003
INA
Global Consortia
Amphibian ARK
USA
2004
INA
BioBankSA
South Africa
2003
INA
Cryo-Brehm
Germany
INA
Stem cellsa; INA
The Frozen Ark
UK
1996
48,000 samples from >5, 500 endangered and non-endangered animal speciesa, b, c, d, e
Exciting developments in cell-based technologies in the past 20 years have provided several potential strategies for the use of somatic cells in biomedical applications, specifically reproductive biology. With the advancement of nuclear transfer and stem cell technologies, somatic cells have the potential to be used directly or indirectly for offspring production. The ability to reprogram differentiated somatic cell nuclei into embryonic or germinal cell lineages has suddenly increased the value, or at least interest, in somatic genome banks. The birth of a gaur (Bos gaurus) in 2000 following transfer of embryos produced by somatic cell nuclear transfer (SCNT) was the first report of an adult fibroblast being used to create offspring from an endangered species (Lanza et al. 2000). Many years later, in September 2011, a new era in somatic cell manipulation was launched when Ben-Nun et al. (2011) reported the production of embryoid bodies derived from induced pluripotent stem cells (iPSCs): the first cases of induced pluripotency in adult fibroblasts derived from endangered species, the silver-maned drill (Mandrillus leucophaeus) and northern white rhinoceros (Ceratotherium simum cottoni).
This chapter provides an overview of the successes and challenges of somatic cell banking and its potential application for reproductive purposes today and in the future. Although the most extensive works have been documented in mammal species, an effort has been made to include the current state of technologies in fishes, birds, reptiles and amphibians.
2 Somatic Genome Preservation
The banking of somatic cells, first and foremost, provides valuable genetic and cellular resource material for basic scientific study, including phylogenomics, evolutionary biology, physiology, and pathobiology, to name a few fields of study. The interest in preserving viable cells is their ability to provide a source of renewable material that can be cultured for long periods, and in some cases, indefinitely. Unlike gametes, somatic cells allow the preservation of the entire genome avoiding dilution of valuable alleles as occurs during fertilization, an important factor when considering species with low numbers of remaining individuals. As well as rapidly increasing the contribution of female genetics in biobanks, somatic cells can be used in cases where collection of viable gametes will not be realized, including individuals: (1) who have died unexpectedly, prior to sexual maturity, or outside of the breeding season; (2) in their prime reproductive years experiencing reproductive dysfunction; (3) in their post-reproductive years who failed or lacked the opportunity to breed; (4) who have been castrated; and (5) whose maturational status is unknown (Mastromonaco and King 2007). The technologies that can be implemented to produce gametes or embryos from somatic cells are discussed in Sect. 3 of this chapter.
2.1 Somatic Cell Collection and Culture
Fibroblasts are one of the common cell types that are banked and have been used for research purposes for over 50 years since Harry Eagle first identified a chemically-defined culture medium that supported cell growth in vitro (Eagle 1955). They have been utilized as primary cultures or continuous cell lines to better understand cellular physiology, investigate a wide range of normal and pathological molecular and biochemical mechanisms, and evaluate cellular response to exogenous stimuli, such as biological substances, environmental toxins and pharmaceutical agents. Although epithelial cells are also widely used and can be derived from tissue explants along with or instead of fibroblasts, the faster-growing fibroblasts can be more easily cultured and sustained in vitro. The benefits of using fibroblasts is that they generally do not require specialized culture systems or cryopreservation protocols and can be isolated from a variety of sources, including organs, such as lung and kidney, muscle and skin. Furthermore, samples from most taxa can be collected with minimal invasiveness (e.g. ear or skin biopsy), opportunistically (e.g. during hoof trim, health check or surgery), and from recently or long-deceased animals. Therefore, unlike gametes and embryos, fibroblasts provide a diploid nucleus and associated cytoplasmic material without the need for species-specific collection, handling, culturing and freezing techniques. Most importantly for the context of this review, fibroblasts have been identified as being one of the most suitable cell types for SCNT (Campbell et al. 2007). An excellent manual for the preparation and culture of fibroblasts and other cell types is Ian Freshney’s Culture of Animal Cells: A Manual of Basic Technique (Freshney 2005). One of the most important criteria for conservation purposes is the establishment of primary cultures from a variety of tissue sources that result in healthy, chromosomally stable and long-lived cell lines.
2.1.1 Mammals
Cell cultures derived from mammals are the most widely used of all the taxa for research and commercial purposes. The American Type Culture Collection (ATCC), the largest biological resource center of its kind in the world, maintains over 3,600 primary and continuous cultures representing over 150 species (www.atcc.org, verified January 20, 2013). In the past 50 years, researchers have established primary cultures from common and endangered breeds or strains of livestock, laboratory animal and companion animal species, including cattle, pig, sheep, rat, guinea pig, horse, dog and cat, but also from a large number of non-domestic and endangered species. San Diego Global Wildlife Conservancy’s Frozen Zoo® is one of the oldest and most diverse repositories of mammalian cell cultures from wild species (www.sandiegozooglobal.org, verified January 20, 2013). Samples representing 20 mammal orders, several hundred species and thousands of individuals have been cryo-banked since 1975 (Houck 2012). Another equally historical biobank, the National Cancer Institute’s repository of over 100,000,000 DNA, tissue and cell line samples, will be integrated into the Smithsonian Institution’s collection later this year (Table 16.1). In 2002, the Mediterranean Marine Mammal Tissue Bank (MMMTB) was established by researchers at the University of Padova, Italy, to collect tissues from stranded and captive marine mammals from the Mediterranean region (Peruffo et al. 2010). Due to the body condition of most stranded animals, the majority of the samples are stored in formalin, however, cultures have been derived from bottlenose dolphin (Tursiops truncatus), sperm whale (Physeter macrocephalus), and striped dolphin (Stenella coeruleoabla) (Peruffo et al. 2010). Other attempts to culture and cryopreserve cells from marine mammals include pygmy sperm whale (Kogia breviceps) (Mancia et al. 2012), Florida manatee (Trichechus manatus latirostris) (Sweat et al. 2001), dolphin (Lagenorhynchus obliquidens) and gray seal (Halichoerus grypus) (Cecil and Nigrelli 1970).
Fibroblast cell establishment and culture techniques in mammals are not complicated and good plating and growth can be obtained following explant or enzymatic digestion and culture at 37 °C in a 5 % CO2 environment (Freshney 2005). Basic culture media, such as Eagle’s or Dulbecco’s Minimal Essential Media (E- or D-MEM), along with the standard supplementation of fetal bovine serum (FBS, 5–20 %) and antibiotics (penicillin/streptomycin or penicillin/streptomycin/fungizone) support cell growth in the primary and passaged cultures (Freshney 2005). Good post-thaw viability of cryopreserved cells can be obtained using a basic freezing medium containing MEM supplemented with 20 % FBS and 10 % dimethyl sulfoxide (DMSO) and a 24 h cooling period at –80 °C in an insulated container prior to immersing in liquid nitrogen. Although researchers have attempted to optimize techniques for specific species or tissue types (León-Quinto et al. 2011), a standardized protocol for mammalian fibroblast culture can be implemented in a laboratory handling a wide variety of species.
Of great importance for wildlife conservation is the ease with which samples can be collected from living mammals without greatly disrupting the animal’s function or health. Skin and ear biopsies obtained using a biopsy punch tool (1-8 mm disposable biopsy punches, Integra-Miltex Inc., Plainsboro, NJ, USA) or biopsy dart (biopsy syringe, Palmer Cap-Chur Inc., Powder Springs, GA, USA) have successfully produced fibroblast cultures from living animals in numerous species (León-Quinto et al. 2011; Torvar et al. 2008; Mastromonaco, unpublished data; Fig. 16.1). A recent study using six threatened Chilean species showed that biopsies can be stored in physiological saline solution supplemented with 1 % antibiotic-antimycotic (ABAM) at 4 °C without altering the time required for primary confluence for up to 7 days for ear punch biopsies and 3 days for skin dart biopsies (Torvar et al. 2008). In our laboratory, on-going studies in domestic and non-domestic bovine species are demonstrating that ear biopsies stored in phosphate buffered saline (PBS) supplemented with 1 % ABAM at 4 °C for 0, 3 and 7 days are capable of producing chromosomally stable primary and passaged cultures (Mastromonaco, unpublished data). Benkeddache et al. (2012) demonstrated that viable cells can be retrieved from rabbit ear biopsies stored in a physiological saline solution supplemented with 10 % DMSO at –20 °C for up to 10 days prior to freezing in liquid nitrogen at similar rates to fresh controls (86 versus 82 %). Samples stored up to 20 days prior to freezing in liquid nitrogen had significantly reduced viability (39 %), but still contained living cells (Benkeddache et al. 2012). These temporary storage conditions are ideal for field collections where access to a laboratory or liquid nitrogen can be hours or days away. If liquid nitrogen or nitrogen vapour are available, biopsies can be stored for a longer period of time prior to culture. Many repositories include pieces of tissue submersed in a freezing medium containing cryoprotectant (e.g. DMEM supplemented with 20 % FBS and 10 % DMSO) and frozen in liquid nitrogen. Work done by researchers at San Diego Zoo Global Wildlife Conservancy, USA, has shown that cultures can be obtained upon thawing of these cryopreserved tissues (M. Houck, personal communication). As with the biopsies from living animals, tissues from deceased animals can provide valuable material hours or even days after their death. Ear fibroblasts are consistently obtained from animals that have died unexpectedly and are found typically within 24-48 h (Mastromonaco, unpublished data).
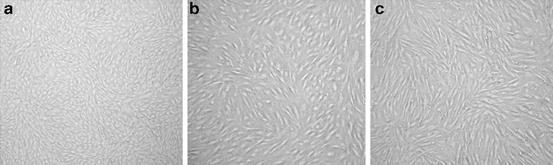
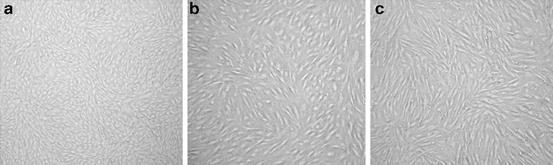
Fig. 16.1
Fibroblast cell cultures at passage 1 derived from different tissue collection techniques. (a) piece of ear tissue; (b) manual biopsy punch; (c) biopsy dart
Several studies have attempted to develop novel techniques for cell preservation. In an effort to reduce cellular injury caused by osmotic stress and improve membrane integrity, researchers used methods of microwave heating (dry preservation; Chakraborty et al. 2008) and spin-drying (lyopreservation; Chakraborty et al. 2011) to promote rapid evaporation of water from living cells. Loi et al. (2008) lyophilized sheep granulosa cells and stored them in the dark at room temperature for 3 years. Upon rehydration, the cells were non-viable and exhibited severe membrane damage, but were able to produce blastocysts when used for SCNT (Loi et al. 2008). Similar results were observed with mouse granulosa cells (Ono et al. 2008) and pig fetal fibroblasts (Das et al. 2010).
2.1.2 Fishes
Commercial stakes in the aquaculture industry have been one of the driving forces behind much of the fish cell culture research. In a recent review, Lakra et al. (2011) reported that fish cells had been cultured and banked from more than 74 species with over 283 cell lines originating from various sources, including organs, embryos and fins. The majority of these cell lines were created for toxicology or disease studies. More recently, researchers interested in preserving rare and endangered fish species or strains have identified the importance of banking fish cell cultures as a source of genetic material to compensate for the difficulty with cryopreserving fish sperm, oocytes and embryos (Rawson 2012). A fish cryobank facility, established in 2008 at the Institute of Biomedical and Environmental Science and Technology, University of Bedfordshire, UK, has already banked tissues and cells from 19 IUCN-listed fish species (Rawson 2012). Cells from the Atlantic sturgeon (Acipenser oxyrhynchus), a species of conservation interest in North America and Europe, have been cultured and banked (Grunow et al. 2011). However, in these cases, the animals were sacrificed as internal organs or larvae were used for culture establishment.
Although many laboratories use mammal-based techniques for fish cell culture, including culture media (e.g. DMEM supplemented with FBS and antibiotics) and incubation in a 5 % CO2 environment (Choresca et al. 2012; Han et al. 2011), fish cells are easy to maintain in air or tightly capped flasks at room temperature without the need for excessive feeding (Lakra et al. 2010; Lannan 1994). Studies indicate that cold-water species grow better between 15 and 21 °C, whereas warm-water species grow better between 25 and 35 °C (Lannan 1994). Bols et al. (2005) indicated that optimal growth for most fish cells lines is well above the natural temperature for the fish. The requirements for pH and ability to grow well in air have led many laboratories to use Leibovitz’s L-15 medium as the basal medium supplemented with FBS and antibiotics (Mauger et al. 2006; Lakra and Goswami 2011).
Many of the available cell lines have been established from internal organs, which can be collected aseptically and are not exposed to surface or environmental microorganisms. However, it is important to develop protocols for live animal collections, as has been done with mammals. Consequently, the use of fins, a highly regenerative tissue, for culture establishment has become very desirable. Whole fins have been used to grow cultures from glass catfish (Kryptopterus bicirrhis) (Han et al. 2011), goldfish (Carassius auratus) (Mauger et al. 2006), and pool barb (Puntius sophore) (Lakra and Goswami 2011), the latter of which grew to 100 passages. The study in goldfish tested cell growth from all fin types and found comparable results in all except the dorsal fin (Mauger et al. 2006). Caudal fin explants have been used to successfully establish cell cultures in the golden mahseer (Tor putitora), an endangered Indian fish species (Prasanna et al. 2000). However, with this tissue source comes an increased risk of microbial contamination and the need to use different or increased concentrations of antibiotics, which often result in increased cytotoxicity and slower or no growth from the tissue explants (Rathore et al. 2007). Fish cultures can be very slow growing (Lannan 1994) sometimes taking up to 3–4 weeks to reach primary confluence (Mauger et al. 2006), which makes it difficult to prevent microbial growth from overcoming cellular growth. Lakra and Bhonde (1996) tested fin culture in eight fish species with unpromising outcomes: only one species attached and grew whereas the other seven either attached but did not grow or were lost to fungal contamination. Studies in our laboratory attempting to optimize culture conditions from fin biopsies of an endangered African cichlid species, ngege (Oreochromis esculentus), have been delayed by persistent culture contamination originating from the tissue samples (Filice, unpublished data). Interestingly, fin biopsy cultures of a commercial tilapia species being used as a model are not succumbing to contamination using the same tissue disinfection, sample processing and culture techniques (Filice, unpublished data). Preliminary attempts at short-term storage of fin samples in a variety of chilled media have not yet yielded promising results (Filice, unpublished data).
2.1.3 Birds
Avian cell lines, primarily from domestic chicken, have been extensively used and studied by virologists and toxicologists for the benefits of both human and avian medicine (Moresco et al. 2010; Portz et al. 2008). The majority of cell culture work in bird species has been done using chicken embryos at an early stage of development (day 8–11). To date, there are very few reports in the literature regarding the preservation of avian genomes as somatic cells. Chinese researchers have been producing early embryo-derived cell cultures to preserve nationally protected domestic chicken breeds, including the Qingyuan partridge chicken (Na et al. 2010), Langshan chicken (Guan et al. 2010) and Big Bone chicken (Su et al. 2011). In all these cases, stable cell lines exhibiting good viability and chromosome normality were created. Recently, Kjelland and Kraemer (2012) used semi-mature and mature feathers containing feather pulp and post-hatch egg shells to culture fibroblasts from six domesticated bird species, including emu (Dromaius novaehollandiae) and ostrich (Struthio camelus). This study is an excellent example of the use of cast-off material and non-invasive sample collection.
The majority of researchers use mammalian-based cell culture systems for the growth of primary and passaged cells as in the studies described above. Minimal essential media supplemented with FBS and antibiotics are generally described. No evidence has been found in the published literature of attempts to optimize culture conditions or cryopreservation techniques in an effort to improve cell viability in other bird species.
2.1.4 Reptiles and Amphibians
Reptiles encompass the least studied taxon when it comes to in vitro techniques, such as cell culture. Lack of pressure from commercial or research interests, particularly health- and food-related industries, has resulted in a smaller number of studies investigating cultured reptile cells. Primary cell cultures have been produced from early embryos of the soft shell turtle (Pelodiscus sinensis) (Liu et al. 2012), heart, liver and muscle of the Chinese alligator (Alligator sinensis) (Zeng et al. 2011), and venom glands of the South American rattlesnake (Crotalus durissus terrificus) (Duarte et al. 1999). Embryos incubated up to 30–40 days were used to establish cultures from green sea turtles (Chelonia mydas), a species listed as endangered by the IUCN (Moore et al. 1997). In an attempt to develop non-lethal techniques for culture derivation, tail and toe clippings were used to prepare cell cultures from five species of Australian dragon lizards (Ezaz et al. 2008). The cell lines were grown for ten passages and retained their viability and normal diploidy. In our laboratory, cell cultures were produced from Komodo dragon (Varanus komodoensis), another IUCN-listed reptile species, from a thin strip of skin taken from the incision site during a surgical procedure (Mastromonaco, unpublished data).
Reptile cells can be grown in mammalian-based culture systems (e.g. DMEM supplemented with FBS and antibiotics) and a 5 % CO2 environment (Stephenson 1966). Studies have shown that although the cells can grow at a wide range of temperatures (Stephenson 1966), most species exhibit optimal growth at 28–30 °C (Ezaz et al. 2008; Moore et al. 1997; Clark et al. 1970). Although studies have investigated the influence of temperature on in vitro cell growth rates, very little work has been done, as in birds, on investigating optimal techniques for culture establishment, culture conditions and cryopreservation methods.
Amphibians, on the other hand, have experienced a recent explosion in biobanking activity. The sudden amphibian crisis resulting from both continued habitat loss and the on-going spread of chytridiomycosis have created urgency among zoological and academic professionals to preserve genetic material from any remaining individuals and species. Fortunately, one of the most widely studied laboratory animal species is the African clawed frog (Xenopus laevis) in which numerous cell lines, primarily from embryos and tadpoles, have been developed for research purposes and thus, some basic tips for amphibian cell culture are available (Anizet et al. 1981). Tadpole hindlimbs were used to produce cell cultures for transgenic studies in a related species, Xenopus tropicalis (Sinzelle et al. 2012). The cell lines were long-lived, surviving over 60 passages, an ideal condition for transgenic studies. There are no descriptions in the scientific literature of cell cultures grown from non-lethal tissue sources or from endangered amphibian species. In November, 2011, Science News Daily reported that researchers at San Diego Zoo Global Wildlife Conservancy had managed to grow cells from frozen biopsy pieces of the endangered Mississippi gopher frog (Rana sevosa), increasing the number of banked individuals to 19 (www.sciencenewsdaily.org, verified January 20, 2013).
Similar to the experience with fish tissues, amphibian samples that cannot be collected aseptically, as with internal organs, are highly susceptible to contamination from the external environment. This is especially the case with early embryos in which the jelly coats harbour microbes encountered during passage through the cloaca and from the environment (Laskey 1970). This poses a challenge when attempting to grow uncontaminated cultures and great effort is spent investigating other antibiotics (e.g. gentamycin) or combinations of antibiotics (e.g. kanamycin + polymyxin E) to increase microbicidal activity (Laskey 1970).
2.2 Ensuring Culture Quality in All Taxa
Material stored in biobanks must be of the highest quality if it is to be considered in the future, particularly for offspring production. Environmental and genetic factors related to the sample itself (species, donor age, tissue type), collection methods, and culture conditions play a role in cell growth and longevity, thereby influencing the viability and normality of the cultures grown in vitro (Mastromonaco et al. 2007). Very few of the published reports detailing the successful establishment of a new cell line characterize the quality of the culture produced either by examining replicative ability or chromosomal stability. A long-lived cell line able to grow for more than 60 passages but with a chromosomal abnormality as with Xenopus tropicalis (trisomic for chromosome 10; Sinzelle et al. 2012) may be adequate for transgenic studies, but not for potential use in offspring production. A similar outcome occurred with common carp (Cyprinus carpio) cells, which grew to almost passage 50, but with only 46–48 % of cells containing a normal chromosomal complement (Lakra et al. 2010) and also with iguana (Iguana iguana) cells, which exhibited minor chromosome changes between passages 10 and 40 (Clark et al. 1970). Likewise, cells that may not have any whole chromosomal concerns, but grow only to passage 1 (Nel-Themaat et al. 2007) will not have the replicative ability to produce the number of cells required for either nuclear transfer or stem cell technologies. Culture establishment techniques that result in primary confluence occurring after more than double the time expected (i.e. >20 days versus 7–10 days) as in the cold stored dart biopsies from Chilean mammal species (Torvar et al. 2008) should be suspect (Fig. 16.2). Replicative ability is correlated with seeding density, specifically the number of viable cells available or able to initiate the culture. The lower the seeding density, the greater the number of mitotic events, and thus, exhaustion of replicative ability, required by each individual cell in order to achieve confluence. Mastromonaco et al. (2006) found that samples obtained by punch biopsy tool or dart resulted in lower quality cultures compared to larger samples of skin or ear collected post-mortem as indicated by decreased lifespan (<30 population doublings versus >50 population doublings), morphological changes characteristic of senescence and increased percentage of chromosomally abnormal cells (up to 58 % versus <20 %). When used for SCNT, short-lived cell lines or those with high levels of chromosomally abnormal cells result in poor blastocyst development as discussed in further detail below.
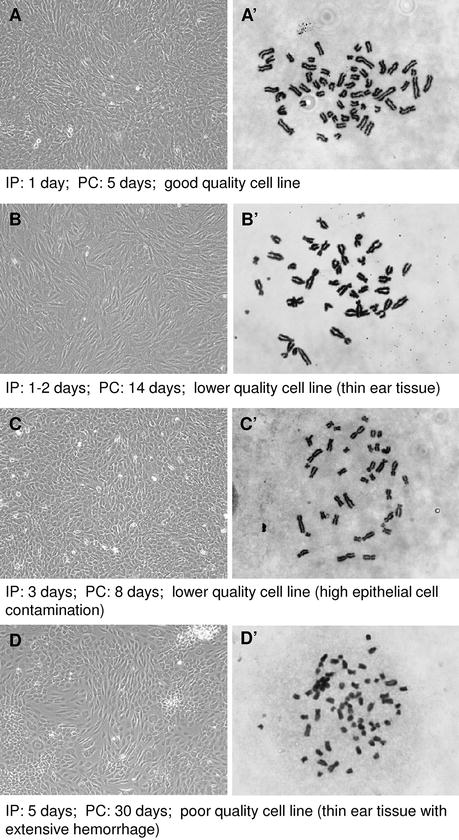
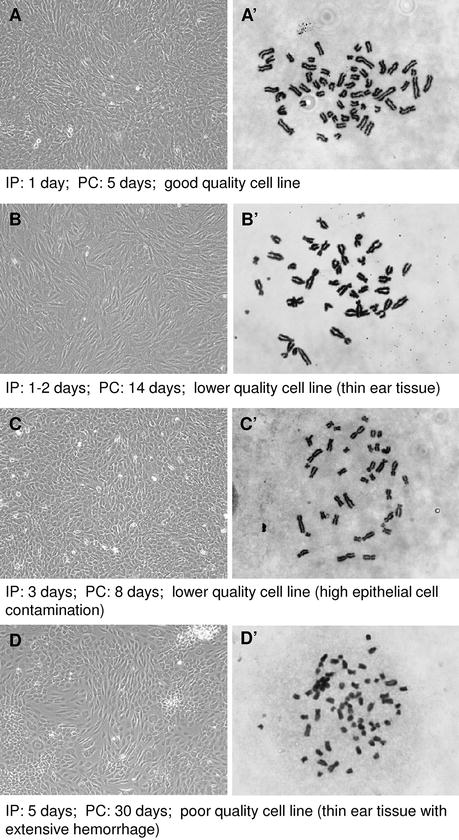
Fig. 16.2
In vitro growth characteristics at passage 1 of cells derived from whole ear tissue. Cell morphology and chromosome spreads from gaur adult (A and A’), Vancouver Island marmot adult (B and B’), cheetah adult (C and C’), polar bear newborn (D and D’). IP number of days for initial plating of dissociated cells; PC number of days to achieve primary confluence
When attempting to biobank from endangered species, particularly from living animals, the samples are generally very small and highly valuable pieces of tissue. Little attention has been given to the need for every explant piece to succeed and grow cells (León-Quinto et al. 2011). It is not generally done, but when banking cells from a genetically valuable individual, tissue explants can be re-plated in a new flask following the initial outgrowth and a further growth of cells can be obtained (Mastromonaco, unpublished data). León-Quinto et al. (2011) tested multiple parameters, including tissue explants versus enzymatic digestion, serum and growth factor supplementation, and cryopreservation media for the Iberian lynx. After assessing 20 different culture conditions and 15 different freezing solutions, the authors identified a protocol promoting increased cell growth and post-thaw viability for the lynx. Although these studies are important for other mammal species, a lot more is known about in vitro cell dynamics in mammals than in other taxa. Greater efforts, primarily time and funding, should be spent investigating these basic parameters for fishes, birds, reptiles and amphibians.
It is standard procedure with cryopreserved sperm samples to carry out a post-thaw evaluation to assess basic sperm characteristics, such as motility, morphology and acrosome membrane status. These data provide an estimate of the fertilizing ability of the sample, necessary information when considering how best to use the sample in the future. Is the sample good enough for artificial insemination or will in vitro fertilization or intracytoplasmic sperm injection be required? A similar approach would be beneficial for banked somatic cell samples whereby a standardized list of criteria are assessed, including post-thaw viability, plating efficiency, number of days to confluence, culture to at least passage 10, and chromosomal content at passage 10. A realistic assessment can then be made and the potential use for the banked cells is known. As seen in cases described previously, cells that may be acceptable for genomic or proteomic studies may not be appropriate for use with techniques leading to offspring production.
Of equal importance as cell viability and normality is the proper identification of the species from which the banked tissues and cell lines are derived. Although necessary for all species, it is particularly important for non-mammalian species where identification based on taxonomic knowledge and phenotypic markers may be more difficult and for samples obtained from field collections compared to well-known captive populations. DNA barcoding, as established by Hebert et al. (2003), is a technique that uses short sections of DNA sequence from a standard part of the genome (e.g. mitochondrial cytochrome c oxidase 1 gene in animals) to differentiate samples at the species level. A recent study by Maya-Soriano et al. (2012) confirmed that the mitochondrial DNA 16S ribosomal RNA sequence that is typically used for DNA barcoding in amphibians was used to successfully identify a variety of banked frog and toad, but not newt, samples to the species level. As the number of “barcoded” species grows and the technique becomes more affordable and accessible, it will become important, if not expected, to include DNA barcoding as one of the required pieces of information on catalogued samples.
2.3 Stem Cell Collection/Production and Culture
The ground-breaking work of scientists at the University of Toronto in the early 1960s (Till and McCulloch 1961) sparked the new research field of stem cell biology. The very nature of these cells, that is, their ability to self-renew and differentiate to produce mature progeny cells (Wagers and Weissman 2004), is what attracted cell biologists and medical professionals to investigate the potential in these cells for tissue repair and regenerative medicine. As described by Wagers and Weissman (2004), stem cells are classified according to their developmental potential, including totipotent (able to give rise to all embryonic and extra-embryonic cell types), pluripotent (able to give rise to embryonic cell types), and multipotent (able to give rise to a subset of cell lineages). Stem cells can also be categorized according to the tissue from which they arise, including embryonic stem cells (from the inner cell mass of the blastocyst), germline stem cells (from gonadal tissue) and adult stem cells (from various sources in newborns to adults). The field of stem cell research has grown dramatically in the past decade for the study of basic biological processes and for application in therapeutic and reproductive medicine. For the purposes of this chapter and the focus on somatic cell banking, embryonic stem cells will not be discussed further. Embryonic stem cell techniques have been reviewed in companion animals by Tayfur Tecirlioglu and Trounson (2007), in birds by Petitte (2006), and in fishes by Hong et al. (2011). It is important to mention, however, that recent advances using mouse embryonic stem cells has resulted in the production of functional sperm (Hayashi et al. 2011) and oocytes (Hayashi et al. 2012).
2.3.1 Germline Stem Cells
Spermatogonial stem cells (SSCs), the basis for continuous sperm production in males, have been investigated as a source of germline material for sperm production from genetically valuable animals. SSC techniques, originally developed in mice, have now been documented in a variety of species (reviewed by Dobrinski and Travis 2007). In the cat and dog, transplantation of SSCs, which involves characterization, isolation, and transfer of cells, has been attempted with mixed results (Travis et al. 2009). In brief, this involved isolating the SSCs followed by transfer into a germ-cell depleted (via radiation) host. On occasion, it has been possible to recover ~20 % of mature sperm cells derived from the donor (Travis et al. 2009). Others have transplanted germ cells from a wild felid (ocelot; Leopardus pardalis) into the domestic cat to produce spermatozoa successfully from the donor (Silva et al. 2012). Recent results in SSC transplantation in primates are encouraging since spermatogenesis can be resumed and lead to the production of fully functional spermatozoa (Hermann et al. 2012). In fishes, fully functional rainbow trout (Oncorhynchus mykiss) sperm were produced by masu salmon (Oncorhynchus masou) following transplantation of trout spermatogonia into the peritoneal cavity of newly hatched salmon embryos (Yoshizaki et al. 2012). These authors document similar successes in a variety of other fish species (reviewed by Yoshizaki et al. 2012). A recent study in birds showed that Japanese quail (Coturnix japonica) spermatogonia were capable of colonizing domestic chicken (Gallus gallus) testes, although the production of fully functional sperm was not detected (Pereira et al. 2012).
In a 2009 review, Tilly and Telfer discuss the exciting new findings supporting the existence of proliferative germ cells in the adult ovary, which may soon challenge the age-old belief that females are born with a non-renewable pool of oocytes at birth. These ovarian stem cells have been isolated from multiple species, including the giraffe (Giraffa camelopardalis), and successfully cultured in vitro (Telfer, personal communication).
2.3.2 Somatic Stem Cells
Adult or somatic stem cells typically arise in tissues with high regenerative capacity, including blood, skin, intestine, and respiratory tract (Wagers and Weissman 2004). Although some of these tissues are difficult to obtain from living animals, especially non-invasively, the banking of a population of cells with an indefinite lifespan and the capacity to create many other cells within the organism, primarily germ cells, is highly enticing and requires further investment in wildlife species. Furthermore, studies using different cell types as nuclear donors for SCNT indicate that the less differentiated the donor nucleus, the greater the developmental potential of the SCNT embryo both pre- and post-implantation (reviewed by Hochedlinger and Jaenisch 2002), suggesting that some adult stem cell populations may be a more appropriate source of donor material for offspring production by SCNT.
The majority of studies on adult stem cells to date have been carried out in laboratory and livestock species, including mouse (brain: Gritti et al. 1996), rhesus monkey (bone marrow: Chang et al. 2006), goldfish (retina: Wu et al. 2001), zebrafish (melanocyte: Tryon and Johnson 2012), pig (skin: Dyce et al. 2004), cattle (bone marrow: Bosnakovski et al. 2005), red deer (antler: Berg et al. 2007), horse (cord blood: Koch et al. 2007), and chicken (feather follicle: Xi et al. 2003). Isolation of adult stem cells may be more difficult than differentiated somatic cells depending on their source as proportionately smaller numbers of stem cells are available in adult tissue fragments. Furthermore, in vitro culture of stem cells ranges from being as effortless as fibroblast culture (mesenchymal stem cells; Cheng et al. 2012) to requiring specialty media (neural stem cells; Hutton and Pevny 2008) and use of feeder cell layers (epithelial stem cells; Nowak and Fuchs 2009). Most importantly, proper characterization of the isolated and cultured cells is required in order to classify them as stem cells, including extended reproductive lifespan, ability to differentiate into other cell lineages, and expression of a specific array of cell surface markers (Dominici et al. 2006).
Despite the claims that biobanking facilities have started including stem cell cultures from endangered species as part of their cryopreserved inventory, very little information is available in the published literature regarding the isolation, establishment and characterization of adult stem cells from wildlife species. Stem cells derived from adipose tissue biopsies of wild Scandinavian brown bears (Ursus arctos) collected during the implantation of tracking devices were shown to differentiate into osteocytes and chondrocytes in vitro (Fink et al. 2011). As with red deer (Cervus elaphus), antler-derived stem cells were cultured from fallow deer (Dama dama) and characterized using specific mesenchymal stem cell markers, such as STRO-1 (Rolf et al. 2008).
Unlike fibroblast cell culture and banking, which can be easily undertaken by most laboratories, adult stem cell culture and banking requires a greater investment in supplies, equipment, and knowledge (e.g. molecular techniques), as well as more stringent criteria for evaluation, which in turn require greater efforts to complete (e.g. long-term culture, exposure to differentiating factors, cell surface marker identification, chromosome analysis). Although samples for adult stem cell culture can be obtained non-invasively, or opportunistically, there is a greater chance of failure to establish a stem cell line compared to fibroblast culture. Therefore, although fibroblast culture banks can become a widespread movement, stem cell banks may continue to remain in specialized centers, most of which are affiliated with academic institutions.
3 Manipulation of Somatic Cells for Potential Offspring Production
The adult somatic cell is genetically identical to the totipotent blastomere from an early embryo, with the exception that numerous modifications to the genome during embryonic and fetal development determined the adult cell’s fate and resulted in a loss in its ability to give rise to multiple cell types, that is loss of pluripotency. However, the presence of the entire genomic code within adult somatic cells makes them an attractive source of genetic material to be considered for propagation of the genetic donor. Researchers have shown that there are multiple strategies for “de-differentiating” somatic cells and inducing an embryonic cell-like state, including nuclear transfer, cell fusion, cell extract exposure, and cell explantation (reviewed by Hochedlinger and Jaenisch 2006). The cytoplasmic machinery within the oocyte has already been proven to reprogram somatic cell nuclei as embryos and living offspring have been produced by SCNT from a wide array of species. Another promising technique still in its infancy is the induction of pluripotency by exposure of cultured somatic cells to cell extracts, virally-mediated transcription factors or other transduction factors. Despite great advances in the past 5–10 years, very little is known about the mechanisms involved in nuclear reprogramming and the potential factors that may influence its efficiency in somatic cells. Many authors agree that an increased understanding of nuclear reprogramming and its control is the only way to enhance the efficiency of techniques, such as SCNT and induction of pluripotent stem cells, in which the goal is to erase all somatic genome modifications and re-establish embryonic genome activity.
3.1 Reproductive Cloning (SCNT)
In the 1950s, nuclear transfer was originally attempted as a method for investigating nuclear equivalence between embryonic and differentiated somatic cells (Briggs and King 1952). Nuclear transfer was, therefore, developed as an experimental tool for the study of specific cellular mechanisms occurring during development. Although it still has great value as an experimental tool, the 1980s brought a shift in the application of nuclear transfer to the propagation of genetically valuable livestock and laboratory animals. The birth of “Dolly” in 1996 came after decades of research by numerous laboratories using a variety of animal models, including frogs, mice, cattle and sheep. Knowing that blastomeres separated from embryos could be reprogrammed to produce viable embryos and offspring, this was the first report of successful reprogramming of an adult somatic cell (from the mammary gland) using SCNT (Wilmut et al. 1997). In the past 16 years since this break-through, hundreds of animals from livestock, companion animal and laboratory animal species have been produced using a variety of somatic cell types and sources. However, extensive efforts in technique development and optimization, and in attempting to understand the cellular machinery that drives successful nuclear reprogramming have greatly improved, but not yet overcome, the high rates of developmental loss that are encountered during all phases of SCNT-related reproductive processes (i.e. during embryo reconstruction, pre-implantation development, post-implantation development and neonatal survival). Fetal and neonatal morphological and physiological abnormalities have been linked to placental aberrations (reviewed by Chavatte-Palmer et al. 2012) and epigenetic defects resulting from altered genome methylation patterns (reviewed by Peat and Reik 2012). Despite these challenges, SCNT has the potential to become a valuable tool for the production of: (1) individuals carrying valuable and/or desirable genetic traits; (2) animal models of disease; and (3) samples for the investigation of the fundamental aspects of pre- and post-implantation embryo development and fetal-maternal interactions (Mastromonaco and King 2007). For endangered species, SCNT offers the possibility of creating embryos from individuals where gametes or germline cells are not available. In more extreme circumstances, SCNT has been contemplated as a method for resurrecting extinct species in which undamaged or complete nuclear genomes are no longer available (see Chap. 19).
3.1.1 Mammals
Mammals have been the most extensively studied taxa for SCNT with live offspring being consistently produced from rodents to primates. The application of SCNT as a method of assisted reproduction for endangered species was first done in mammals (gaur (Bos gaurus); Lanza et al. 2000) and has yet to be reported in any other taxa. Researchers faced with the task of obtaining large numbers of oocytes required for SCNT from endangered females overcame this challenge by using oocytes from related domestic species as cytoplasmic recipients; a technique called interspecies SCNT (iSCNT). This idea was supported by the study of Dominko et al. (1999), which demonstrated that the bovine cytoplasm was capable of reprogramming, at least partially, diverse donor nuclei (sheep, pig, monkey, rat) past maternal-embryonic transition and, with the exception of the rat, progress to the blastocyst stage. Other than a handful of studies on non-traditional, albeit domesticated or laboratory-based, species using intraspecies SCNT, including dromedary camel (Camelus dromedarius; Wani et al. 2010), ferret (Mustela putorius furo; Li et al. 2006b), rhesus monkey (Macaca mulatta; Zhou et al. 2006), and long-tailed macaque (Macaca fascicularis; Ng et al. 2004), all reports in wild species involve iSCNT. To date, published reports show that more than 30 wild species have been attempted with the majority of the species achieving blastocyst development, but poor post-implantation success (Table 16.2; Fig. 16.3).
Table 16.2
Overview of interspecies SCNT outcomes
Cell donor | Cell type | CITES appendix | Recipient oocyte | Reconst. embryos (n) | Blastocysts (%) a | Transferred embryos (n) | No. fetuses/pregnancies (n) | Off-spring (n) | Outcome | Reference | ||
~D30 | ~D60 | ~D120 | ||||||||||
Bovidae | ||||||||||||
Bos gaurus (Gaur) | Skin fibroblast | I | Bos taurus | 922 | 33.2–37.5 | 106 | 4 | 3 | 1 | Dead <24 h | Srirattana et al. (2012) | |
Bos gaurus (Gaur) | Ear fibroblast | I | Bos taurus | 228 | 18.1 | ND | ND | ND | ND | Mastromonaco et al. (2007) | ||
Bos gaurus (Gaur) | Skin fibroblast | I | Bos taurus | 692 | 12.0* | 44 | 6 | 2 | 1 | Dead <1 week | Lanza et al. (2000) | |
Budorcas taxicolor (Takin) | Ear fibroblast | II | Bos taurus | 227 | 6.6 | ND | ND | ND | ND | Li et al. (2006a) | ||
Taurotragus oryx (Common Eland) | Seminal epithelial cell | Not listed | Bos taurus | 209 | 0 | ND | ND | ND | ND | Nel-Themaat et al. (2008) | ||
Naemorhedus goral (Himalayan Goral) | Skin fibroblast | I | Bos taurus | 506 | 0–5.0 | ND | ND | ND | ND | Oh et al. (2006) | ||
Bos javanicus (Banteng) | Ear fibroblast | Not listed | Bos taurus/Bos indicus | 319 | 20.0–28.0 | 38 | 2 | 0 | 0 | 0 | Sansinena et al. (2005) | |
Capra ibex (Alpine Ibex) | Ear fibroblast | Not listed | Capra hircus | 790 | 11.0 | ND | ND | ND | ND | Wang et al. (2007) | ||
Capra pyrenaica pyrenaica (Pyrenean Ibex) | Ear fibroblast | Extinct | Capra hircus | 782 | NA | 184 | 7 | 1 | Dead <24 h | Folch et al. (2009) | ||
Pantholops hodgsonii (Tibetan Antelope) | Ear fibroblast | I | Oryctolagus cuniculus | 1097 | 5.5-20.4 | ND | ND | ND | ND | Zhao et al. (2006) | ||
Tragelaphus eurycerus isaaci (Bongo) | Skin fibroblast | III: Ghana | Bos taurus | 365 | 14.0-24.2* | ND | ND | ND | ND | Lee et al. (2003) | ||
Ovis canadensis mexicana (Desert Bighorn Sheep) | Skin fibroblast | II: Mexico | Ovis aries | 265 | NA | 223 | 5 | 0 | 0 | 0 | Williams et al. (2006) | |
Ovis orientalis musimon (European Mouflon) | Granulosa cell | I | Ovis aries | 23 | 30.4* | 7 | 2 | 1 | 1 | 1 | Live | Loi et al. (2001) |
Ovis ammon (Argali Sheep) | Skin fibroblast | II | Ovis aries | NA | 0 | 28 | 1 | 0 | 0 | White et al. (1999) | ||
Ovis orientalis isphahanica (Esfahan Mouflon) | Skin fibroblast | I | Ovis aries | 667 | 7.6 | 12 | 2 | 2 | Dead <24 h | Hajian et al. (2011) | ||
Pseudoryx nghetinhensis (Saola Antelope) | Skin fibroblast | I | Bos taurus | 312 | 35.1 | ND | ND | ND | ND | Bui et al. (2002) | ||
Bison bison athabacae (Wood Bison) | Ear fibroblast | II | Bos taurus | 226 | 19.2 | ND | ND | ND
![]() Stay updated, free articles. Join our Telegram channel![]() Full access? Get Clinical Tree![]() ![]() ![]() |