22 Snakehead Rhabdovirus University of Maine, Orono, USA The focus of this chapter is snakehead rhabdovirus (SHRV), a Novirhabdovirus, in the family Rhabdoviridae. We will present a history of this virus in the natural host and provide an overview of the current state of knowledge about this virus family, with particular focus on SHRV. Rhabdoviruses have a wide host range and can infect plants, insects and vertebrates, including humans and other mammals. A number of rhabdoviruses are pathogens that are responsible for causing devastating economic losses to the finfish industry. Several rhabdovirus species infect fish (see Table 22.1). In our laboratory, we are using the SHRV infection of zebrafish (Danio rerio) to model fish rhabdoviral infections and the subsequent host innate immune response. With the molecular reagents, transgenic animals and genomic data available for the zebrafish system, this model species can provide critical insight into the mechanisms of viral pathogenesis. SHRV was isolated from striped snake-head fish during an outbreak of epizootic ulcerative syndrome (EUS) (Ahne et al., 1988; Wattanavijarn et al., 1986). EUS is a seasonal, freshwater and estuarine disease of ambiguous aetiology that has been identified in numerous fish species (>100) from Asia, Australia, North America and Africa. Outbreaks of EUS have had dramatic impacts on regional fisheries, threatening local economies and food supplies (Oidtmann, 2012). EUS typically manifests itself in affected fish through skin lesions that lead to subsequent infection of underlying musculature and viscera. While the exact mode(s) of infection remain controversial, it is generally accepted that fish afflicted with EUS retain a common pathological feature of ulcerative lesions containing fungal hyphae (usually the ubiquitous Aphanomyces invadans, also known as A. piscida) that elicit granulomatous responses (Baldock et al., 2005). The most widely held belief is that A. invadans is the primary causative agent of EUS outbreaks and that these infections are thought to be facilitated by environmental factors, such as infection by viruses like SHRV or cutaneous damage. Some investigators hold that EUS represents a constellation of polymicrobial infections involving viruses (like SHRV), bacteria and fungi, including, but not always, A. invadans, that are triggered by environmental insults (e.g. pH, temperature, salinity, mechanical, infection) (Baldock et al., 2005; Oidtmann, 2012). It is critical to emphasize that while viruses like SHRV are thought to participate to some degree in EUS, they are not the principal causative agent. Table 22.1. Fish rhabdoviruses. Selected references (1986–2010) on rhabdoviruses that afflict fish species. Members of the Vesiculovirus genus and the Novirhabdovirus genus are shown. Despite this, many previously undescribed viruses (Frerichs et al., 1986; Hedrick et al., 1986; Ahne et al., 1988; Wattanavijarn et al., 1988; Subramaniam et al., 1993; Roberts et al., 1994; Frerichs, 1995; John et al., 2001) have been isolated from fish during EUS outbreaks, and these have provided critical insights into fish immunology. Numerous rhabdoviruses have been isolated from EUS-affected fish. Frerichs et al. (1986) was the first to report a single virus type that had been isolated from multiple species of fish across a broad geographic area (Thailand and Burma). This virus, derived from wild and pond-culture striped snakehead fish, as well as freshwater eels, formed a bullet-shaped particle with a typical rhabdoviral structure (120 ± 10 nm × 80 ± 5 nm). The virus was subsequently identified as ulcerative disease rhabdovirus (UDRV). Ahne et al. (1988) later characterized a similar rhabdovirus that had been isolated from striped snakehead fish by Wattanavijarn and Wattanodorn (1986) following an EUS outbreak in Thailand. This virus came to be known as SHRV, and serological analysis revealed no relationship to five other reference fish rhabdoviruses. Kasornchandra et al. (1992a) undertook a more intensive comparative study of the rhabdoviruses isolated by Wattanavijarn et al. (1986) and Frerichs et al. (1986, 1989). SHRV was found to have a bacilliform morphology and ranged in size from 180 to 200 nm long and 60 to 70 nm wide (Kasornchandra et al., 1992a). An analysis of the structural proteins by SDS-PAGE revealed a pattern similar to infectious haematopoietic necrosis virus (IHNV), viral haemorrhagic septicaemia virus (VHSV) and hirame rhabdovirus (HIRRV) (Kasornchandra et al., 1992a). The two UDRV particles that were characterized (UDRV-BP and UDRV-19) were found to have bullet-shape morphology and ranged in size from 110 to 130 nm long and 50 to 65 nm wide. SDS-PAGE revealed that the structural proteins possessed by UDRV-BP and UDRV-19 isolates were most similar to spring viremia of carp virus (SVCV) and pike fry rhabdovirus (PFRV) (Kasornchandra et al., 1992a). Serological comparisons demonstrated that while they were both isolated from snakehead fish, SHRV and the UDRV viruses were distinct rhabdoviruses, although UDRV-19 and UDRV-BP appeared to be identical. Subsequently, Lilley and Frerichs (1994) characterized a novel rhabdovirus, known as T9204, which had been isolated from striped snakehead. While bearing close similarity to SHRV, it differed in several ways and thus was determined to be another novel rhabdovirus strain associated with EUS. Lio-Po et al. (2000) identified 91-97 as yet another rhabdoviral isolate from samples collected from snakehead during an EUS outbreak in the Philippines in 1991. The 91-97 virus exhibited different serological properties, which indicated that it was antigenically distinct from SHRV and more closely related to UDRV. SHRV replicates at an optimum temperature range of 24–30°C. SHRV, like other rhabdoviruses, is an enveloped, non-segmented, negative-sense, single-stranded RNA virus. The virus is built around the negative-sense RNA genome, which is approximately 11,000 nucleotides (11 kb) long. Rhabdoviruses in general have five genes that are organized in a linear arrangement. Novirhabdoviruses are unique in that they have six genes, with an additional nonvirion (NV) gene between the glycoprotein (G) and polymerase (L). The small genome has an unmodified 5′-triphosphate (5′-ppp) group and 3′ hydroxyl ends and is comprised of genes with the gene order (3′-N-P-M-G-NV-L-5′). The first four nucleotides at the 3′-ends of SHRV, IHNV, HIRRV and VHSV are identical (Kim et al., 2005). The gene order is conserved among Rhabdoviruses and between the genes there are short inter-genic regions (Purcell et al., 2012). Briefly, the nucleoprotein (N) encloses the RNA; the phosphoprotein (P) is a co-factor for the RNA polymerase and a chaperone for N; the matrix (M) protein is critical for virus assembly and budding; the G is a transmembrane protein responsible for attachment to target cells and facilitates membrane fusion; and the L is the viral RNA polymerase (Rieder and Conzelmann, 2011). Among fish rhabdoviruses, the L protein of SHRV shows the highest sequence homology to HIRRV, IHNV and VHSV (Kim et al., 2005). The ratio of gene expression is regulated during transcription. For instance, there is a polarity of transcription that starts at the 3′ end of the virus genome to the 5′ end, and results in more mRNA for proteins encoded in the 3′ end of the genome than for proteins located at the 5′ end (Cann, 1993; Rieder and Conzelmann, 2011). The entire lifecycle of SHRV occurs in the cytoplasm of the infected cell and replication is carried out by the viral RNA polymerase. Packaging of this enzyme within the virus nucleocapsid is essential because no known hosts contain enzymes capable of decoding and copying the RNA genome (Cann, 1993). As a negative-sense RNA virus, it contains virion RNA that is complementary to mRNA, which must be copied into the complementary positive sense mRNA before viral proteins can be made. The newly transcribed negative strands may be used as templates for a full-length negative-sense genome. SHRV enters its target cell via receptor-mediated endocytosis. This process is mediated by interaction of the viral G protein with a cell surface receptor. The G protein is synthesized by ribosomes bound to the endoplasmic reticulum and is transported and inserted into the plasma membrane. These trimeric proteins are critical for assembly into virions, and virus entry, because G proteins promote fusion of the viral and host endosomal membranes, after which the viral nucleocapsid is released into the cytoplasm of the host cell. Fish rhabdoviruses were first assigned to either the genus Vesiculovirus or the genus Lyssavirus based on their protein profiles following gel electrophoresis. Mammalian viruses such as rabies virus and vesicular stomatitis virus also fall into these genera. Additional accepted genera include: Ephemerovirus, Cytorhabdovirus, Nucleorhabdovirus and Novirhabdovirus (Hoffman et al., 2005). Molecular phylogenies have been determined for the Rhabdoviridae family using N, G or L gene sequences. It should be noted that a general phylogenic tree cannot be constructed based on the G gene due to low sequence identities, and sequences for the L gene are not available for all of the species of each genus. A phylogenetic tree of the Rhabdoviridae family has been constructed using sequences of the N gene, which is reasonably conserved (Hoffman et al., 2005). The phylogenetic analysis confirmed the established classification of rhabdoviruses into six genera. Based on complete N gene sequences, fish viruses fall within the Vesiculovirus and Novirhabdovirus genera (Hoffman et al., 2005) (see also Table 22.1). Species within the Novirhabdovirus genus contain nucleotide sequences that are similar among all identified members of the genus (e.g. phylogenetic clustering), but are distinct from rhabdoviruses of the other genera (Hoffman et al., 2005). Of interest, all members of this genus are fish pathogens. The gene is conserved among all of the virus species within this genus, implying that NV may play a significant role in viral pathogenesis (also see section 22.5). Fish rhabdoviruses, in general, cause disease that is commonly characterized by acute haemorrhagic septicaemia (Purcell et al., 2012). The disease manifestation impacts multiple organs and clinical symptoms such as skin darkening, ascites and exopthalmia can be observed, although it should be noted that varied forms of disease have been described for IHNV and VHSV (Purcell et al., 2012). As mentioned above, SHRV has been associated with EUS (Wattanavijarn et al., 1986; Ahne et al., 1988). Upon further characterization, researchers have found that EUS is a complex disease process that seems to involve mechanical, environmental and pathological insults. Oidtmann (2012) provides a thorough review of the clinical and diagnostic features of EUS. Briefly, fish affected by EUS develop clinical signs that are often indistinguishable from other conditions. Typical EUS symptoms are non-specific in nature and range from mild reddening of the skin to severe ulcerations. Histopathologically, fish exhibiting signs of EUS often, but not always, form mycotic epithelial granulomas as a result of an infection of the muscle tissue. In some circumstances, the presence of multinucleated giant cells, in the context of other factors, are indicative of EUS. In other instances, EUS fish succumb to infections prior to the display of these signs of a robust inflammatory response. These EUS clinical symptoms differ from those observed in experimental SHRV infections in zebrafish, in which discrete pathological features were observed, and in snakehead fish, where no obvious EUS-like lesions were noted following challenge by immersion or intraperitoneal injection. SHRV may contribute to EUS but does not itself cause EUS. The clinical symptoms observed are triggered by infection with A. invadans. Our laboratory has previously reported the successful infection of zebrafish embryos and adults with SHRV (Phelan et al., 2005b). Pathological effects were observed in zebrafish infected with SHRV, and the infection elicited an antiviral innate immune response (discussed in detail in section 22.6). Experimental infection of both embryonic and adult zebrafish led to infection kinetics and histopathology indicative of acute infection. Gross pathology was observed in adult zebrafish injected intraperitoneally with SHRV. Fish were examined 2 days post infection with SHRV for signs of viral infection and clinical disease. Moribund fish showed erratic swimming patterns and lingered near the surface of the water. Adults also exhibited severe petechial haemorrhages on the abdomen compared with control fish, which exhibited no erratic behaviour or lesions (Phelan et al., 2005b). Histological examination was conducted on both embryonic and adult zebrafish from infected and control groups. First, blood vessels, branchial regions and livers of control and infected embryos and juveniles were compared (Phelan et al., 2005b). Histopathology included high numbers of monocytes in the blood vessels of the perianal region, marked absence of erythrocytes, high numbers of mucus cells in the buccopharyngeal epithelium and necrotic tissue in the pharyngeal epithelium itself. In addition, pigment cells of infected fish appeared to be irregular in shape compared with the pigment cells of control fish. The lumen of the swim bladder was congested with cell debris in infected fish, and the livers of infected fish also contained necrotic cells. The liver tissue also displayed cytoplasmic vacuolization and pyknosis of hepatocytic nuclei, indicating toxic conditions. Lastly, the hepatic ducts were obstructed in the infected fish but not in the control fish. Histopathology of adult zebrafish infected with SHRV by intraperitoneal injection was also observed. These findings were in agreement with the gross pathology observed in adults described above. Fig. 22.1 shows comparisons of the scales and epidermis (Fig. 22.1A,B) and ovaries (Fig. 22.1C–E) of control and SHRV-infected adult zebrafish (Phelan et al., 2005b). The infected adult fish presented subepidermal petechial haemorrhages and oedema near the site of injection (Fig. 22.1B), compared with PBS-injected control fish, which showed no signs of infection or inflammation. Females infected with SHRV displayed degeneration of secondary oocytes (Fig. 22.1D,E), such as disruption of the yolks of the secondary oocytes and subsequent reabsorption of the yolk by neighbouring epithelial granulose cells. Further, infected fish also exhibited fluid and inflammatory cell accumulation in the abdominal cavity. Histopathology associated with infection by other Novirhabdoviruses is similar. In hirame (HIRRV), histological analysis shows necrotic changes in the kidney and spleen (Oseko, 1992; Roberts, 2012). Externally, hirame present with abdominal distension. In fish afflicted with VHSV (e.g. rainbow trout), necrosis of haematopoietic tissue is observed, as well as the presence of degranulated macrophages (Roberts, 2012). Further, fish infected with the North American strain of VHSV also displayed histological evidence of necrosis in the kidney and pancreas (Meyers et al., 1999). Clinical signs of infection of rainbow trout infected with IHNV also include abdominal distension, abnormal behaviour, darkening of the skin and haemorrhage. Histological observations show that necrosis is common in the kidney and spleen (Romero et al., 2005). Members of the genus Novirhabdovirus are distinguished by the presence of a non-virion (NV) gene located between the G and L genes. In most rhabdovirus genomes, over 90% of the nucleotides are protein-coding, making it reasonable to assume that the NV gene must be critical to the virus, but the function remains unknown (Johnson et al., 2000). Studies attempting to characterize a functional role for NV in the pathogenesis of SHRV have led to controversial results. Studies with SHRV have suggested that NV has no critical function in SHRV replication, nor is it required for SHRV pathogenesis (Johnson et al., 2000; Alonso et al., 2004). Others have demonstrated that in IHNV, the NV protein causes cell rounding and therefore interacts with host cytoskeletal elements (Chiou et al., 2000). Recent studies have indicated roles for NV using two separate Novirhabdoviruses. One study using recombinant IHNV lacking the NV gene found that NV may function to limit the host interferon (IFN) response in fish because the recombinant virus led to greater transcription of the rainbow trout Mx and IFN1 in vitro and had higher levels of functional IFN (Choi et al., 2011). Recently, studies have also demonstrated that the NV protein of VHSV delays the onset of apoptosis in cell culture, as recombinant VHSV lacking NV induced apoptosis sooner than WT VHSV (Ammayappan and Vakharia, 2011). These reports imply that there are inconclusive results about the role of NV in pathogenesis and whether it is virus-specific. Fig. 22.1. Histopathology of adult zebra fish infected with SHRV by intraperitoneal injection. (A) Normal scales and epidermis of control fish. Magnification 100×. (B) Scales and epidermis of infected fish. Black arrowheads indicate subdermal oedema and haemorrhaging. The white arrowhead indicates haemorrhaging in the underlying muscle tissue. Magnification 100×. (C) Ovaries of control fish showing normal egg development, with generations of ova in different developmental stages. The white asterisk indicates a primary oocyte, the black asterisk indicates a secondary oocyte, and the black arrowhead indicates the epithelial granulosa (nursing) cells. Magnification 200×. (D) Degenerating secondary oocyte of SHRV-infected fish (black asterisk). Primary oocytes seem to be unaffected (white asterisk). Magnification 200×. (E) Epithelial granulosa cells (white arrows) reabsorbing remaining yolk from secondary oocyte. (Previously published in Phelan et al. (2005).) The vertebrate host immune system is comprised of two branches, the innate and adaptive immune systems. The innate and adaptive arms of immunity work synergistically to mount a robust immune response against invading pathogens. Innate immunity is essential for the first line of host response to foreign molecules that have not been previously encountered. It is intrinsic to all eukaryotes and active early in development. Adaptive immunity is restricted to organisms ranging from jawed fish to mammals and gains full competence later in development. The adaptive immune response initiates a specific response against pathogens through recognition of pathogen-specific epitopes. This branch of immunity also provides host immunological memory (Akira, 2009). The adaptive immune system generates a range of antigen receptors (the T- and B-cell receptors) through DNA rearrangement. Once a pathogen is encountered, lymphocytes with the appropriate antigen receptor to that pathogen eliminate the pathogens in the late stage of infection. All vertebrates possess the components necessary for an adaptive immune response (reviewed in Trede et al., 2004; Guo et al., 2009; Boehm et al., 2012). However, it is known that production of neutralizing antibodies induced by infection or vaccination is important for long-term adaptive immunity to fish rhabdoviruses (Purcell et al., 2012). In addition, fish B cells show phagocytic behaviour (Li et al., 2006), and have a wide range of T-cell associated genes that are upregulated after rhabdoviral infection (Castro et al., 2011). A recent report shows progression towards understanding the fish complement system (Nakao et al., 2011), but the details defining which complement components are critical for virus neutralization remain to be elucidated. By contrast, innate immunity discriminates self and non-self using germline-encoded receptors called pattern recognition receptors (PRRs). PRRs recognize molecular patterns of components specific to microbial organisms (e.g. single-stranded RNA). Nucleic acids are the primary viral component recognized by the immune system. Protection from rhabdoviral infection depends on innate immunity, in particular the interferon (IFN) system that is rapidly induced upon infection. Teleosts such as zebrafish possess the components critical for mounting an innate and adaptive immune response, similar to that found in other vertebrates. The innate immune system is the first line of defence against invading organisms. It is composed of cells and molecules that defend the host from infection by pathogens. In contrast to the adaptive branch of immunity, it does not confer protective memory to the host. The innate immune response is also critical for mounting a robust adaptive immune response. Pathogens are detected by recognition of molecules such as pathogen-associated molecular patterns (PAMPs). Pattern recognition receptors (PRRs) are essential for recognition of PAMPs and generation of immune responses. Activation of signal transduction pathways stimulated by PRR leads to the production of antiviral effector molecules, in particular the type I IFN system. Recognition of rhabdoviruses in fish is postulated to be similar to that observed in mammals (Zou et al., 2010; Palti, 2011; Purcell et al., 2012). The Toll-like receptors (TLR) are perhaps the best characterized PRRs. They are an evolutionarily-conserved family of PRRs capable of recognizing a broad range of PAMPs, including viral single- and double-stranded RNA, as well as unmethylated CpG motifs. All TLRs, in vertebrates, possess an extracellular domain containing leucine-rich repeats (LRR), a transmembrane domain and a cytoplasmic Toll/interleukin-1 (IL-1) receptor (TIR) domain (Akira, 2009; Akira and Takeda, 2004). Upon recognition of PAMPs, TLRs recruit adaptor proteins like myeloid differentiation primary response gene (88) (MYD88), MYD88 adaptor-like (MAL), Toll-like receptor adaptor molecule 1 (TICAM1) and TICAM2, and initiate signal transduction pathways (Kumar et al., 2009) (Fig. 22.2A) that mediate inflammatory responses. Note that MAL is also known as toll-interleukin 1 receptor (TIR) domain-containing adaptor protein (TIRAP), TICAM1 is also known as TIR domain-containing adaptor-inducing interferon-β (TRIF) and TICAM2 is also known as TRIF-related adaptor molecule (TRAM). In mammals, TLR3, TLR4, TLR7, TLR8 and TLR9 can initiate antiviral immune responses. TLR3 recognizes double-stranded RNA and uses a MYD88-independent, TICAM1-mediated pathway to trigger an antiviral response. TLR4 has been shown to be activated by several viruses, including respiratory syncytial virus (RSV) (Kurt-Jones et al., 2000) and herpes simplex virus-1 (HSV) (Villalba et al., 2012). It uses both MYD88-dependent and -independent pathways to initiate immune responses. The principle agonists of TLR7 and TLR8 are single-stranded RNA, while TLR9 recognizes unmethylated CpG oligonucleotide (ODN) sequences. All three of these mammalian TLRs use MYD88-dependent pathways to mount antiviral responses. Fish also possess Tlr proteins that mediate potent immune responses; however, after multiple rounds of genome duplication many of the tlr genes that are present likely recognize alternative PAMPs (Sullivan et al., 2009). For example, a thorough examination of gene histories indicates that zebrafish possess tlr4 genes that are paralogous to mammalian TLR4 genes (Sullivan et al., 2009). Additionally, zebrafish appear to lack genes homologous to mammalian CD14 and MD2. When exposed to the mammalian TLR4 ligand LPS, Tlr4a and Tlr4b proteins appear unresponsive, failing to activate an NF-κB reporter. Further, fish appear to lack a TICAM2 ortholog, which is critical for mediating a MYD88-independent, TICAM1-dependent antiviral response in mammals (Sullivan et al., 2007). The most parsimonious explanation is that ticam2 was lost in teleosts following the divergence of the rayfin and lobefin fishes 450 million years ago. The zebrafish Ticam1 protein has evolved unique structural features that have had important functional impacts on signaling. For example, zebrafish Ticam1 protein, unlike mammalian TICAM1, does not recognize Traf6, due to the lack of a functional Traf6 binding motif. Ticam1-mediated NF-κB activation is triggered through its interaction with Rip1. Additionally, Ticam1 triggers an antiviral interferon response through an Irf3/7-independent mechanism. Zebrafish Ticam1 can interact with Tlr3, which appears to have retained its function through evolution. When Tlr3 is overexpressed, it triggers an NF-κB-dependent immune response (Phelan et al., 2005a). When zebrafish are exposed to SHRV, Tlr3 expression is upregulated. In fugu, Tlr3 is responsive to the dsRNA analogue poly I:C, triggering the activation of an interferon luciferase reporter construct (Matsuo et al., 2008). A novel Tlr22 protein was also discovered in fugu and found to be even more responsive to poly I:C stimulation. Unlike Tlr3, which exhibits restricted expression in endosomes, Tlr22 is expressed on the cell surface. Together, Tlr3 and Tlr22 are thought to provide a more comprehensive defence to viral infection through their combined recognition of dsRNA. In addition to poly I:C stimulation, fish have shown responsiveness to ligands shown to activate other mammalian TLRs. For example, Atlantic salmon can elicit antiviral responses to mammalian TLR7 ligand imidazoquinoline S-27609 (Kileng et al., 2008). It is important to note that conclusive evidence needs to be obtained to establish direct relationships between fish Tlr proteins and known mammalian agonists. Despite considerable sequence homology, gene histories must be investigated and functional assays must be performed in order to prove conservation of Tlr function. The zebrafish model system offers opportunities to bridge the gap between human health and fisheries health because of the wealth of tools and information that are available. Using the zebrafish system, it is possible to interrogate a fish genome to explore questions of the gene history and then perform intensive assays aimed at answering important biological questions that can broadly expand our understanding of fish health (Sullivan et al., 2007, 2009; Sullivan and Kim, 2008).
22.1 Introduction and Overview
22.1.1 History: isolation of rhabdovirus, including SHRV
22.2 Snakehead Rhabdovirus Genome Structure and Life Cycle
22.3 Phylogeny of Rhabdoviruses
22.4 Pathogenesis: Clinical Symptoms
22.4.1 Clinical symptoms of EUS
22.5 Pathogenesis and Clinical Symptoms: SHRV
22.6 Pathogenesis: Immune Response to SHRV
22.6.1 Innate immune response to SHRV
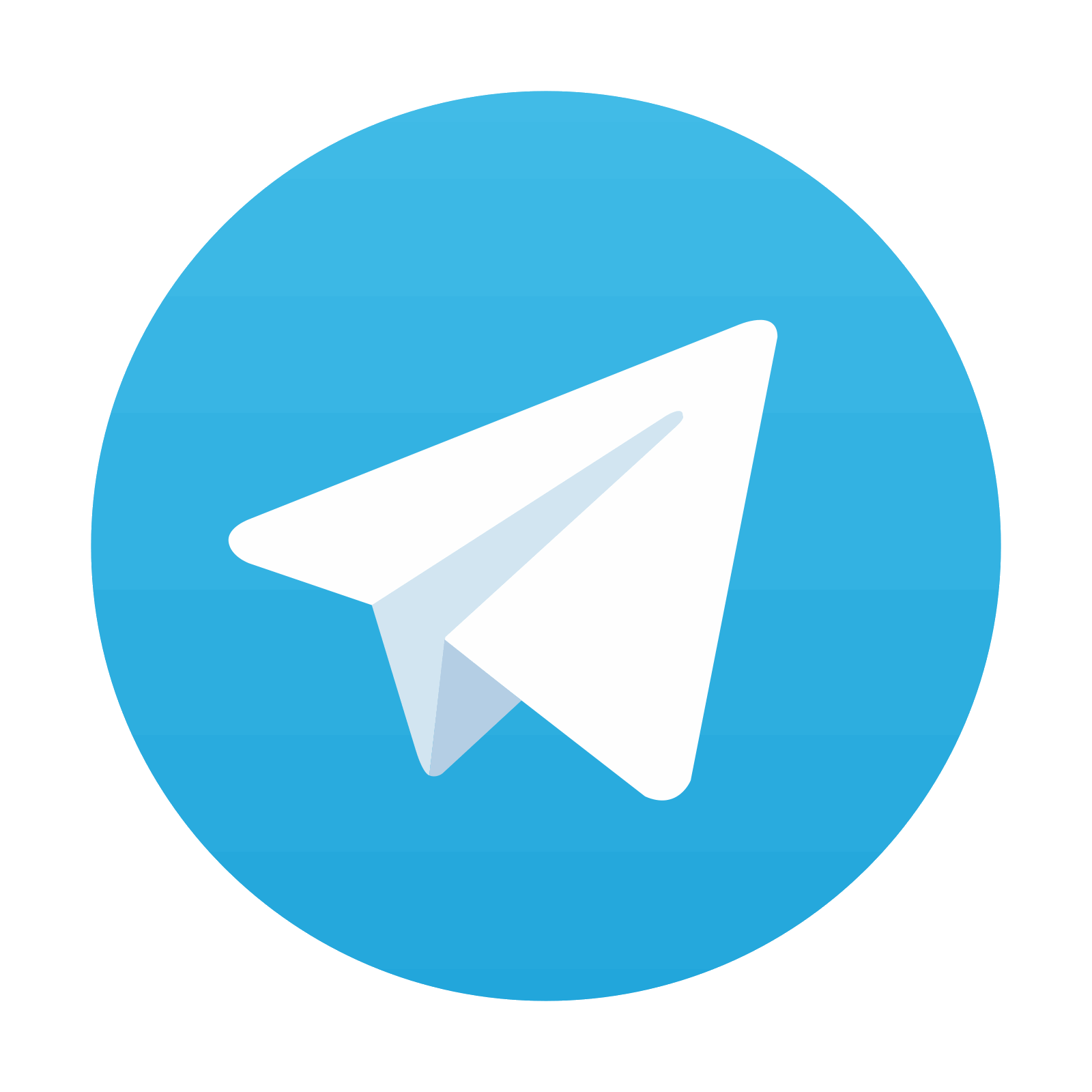
Stay updated, free articles. Join our Telegram channel
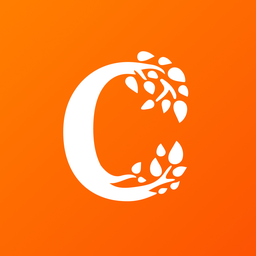
Full access? Get Clinical Tree
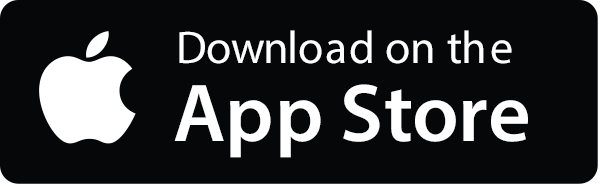
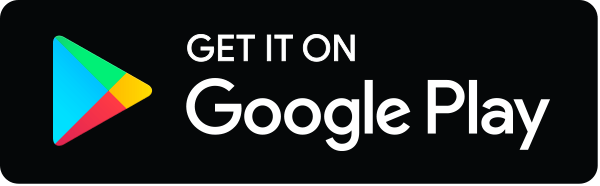