Catherine M. Creighton and Leigh A. Lamont Department of Companion Animals, Atlantic Veterinary College, University of Prince Edward Island, Charlottetown, Prince Edward Island, Canada Sedatives and tranquilizers play an important role in day‐to‐day veterinary practice. The use of these medications as part of an anesthetic plan has many advantages, including, but not limited to, calming the patient, facilitating intravenous (IV) catheterization, providing analgesia, blunting sympathetic responses to surgical stimulation, reducing anesthetic requirements, and promoting smooth induction and recovery. The selection of drugs should be individualized to the patient. Species, age, patient temperament, pathologic processes, blood volume status, and so on should be considered when selecting drugs and doses. The distinction between a tranquilizer and sedative is often nebulous due to species and dosing differences. For example, α2‐adrenergic receptor agonists are effective in both horses and cattle, but cattle may require one‐tenth the horse dose to achieve a comparable level of sedation. Phenothiazines are efficacious in dogs and horses but not so in swine. These distinctions are discussed in this chapter and in chapters pertaining to techniques in different species. In general, a tranquilizer induces a feeling of calm (anxiolysis). Sedatives, while reducing anxiety, also reduce the overall response to external stimuli. Analgesia is a feature of some sedatives but certainly not all. Clinical practice demonstrates that different species can vary widely in their response to a class of drug, and individuals within a species may also display variable effects. Terminology used varies depending on the source but, in this chapter, all the medications are termed “sedatives,” knowing that effects will vary with species and dose. There are a variety of classes of sedatives currently used in veterinary medicine. Phenothiazines, benzodiazepines, and α2‐adrenergic receptor agonists are among the most commonly used in domestic animals, and they are often combined with opioids and/or other injectable agents to produce variable states of calmness, sedation, and possibly analgesia depending on the medications used. In dogs and cats, increasing appreciation of the stress associated with veterinary visits has resulted in a significant increase in the use of off‐label anti‐anxiety medications such as gabapentin, trazodone, and melatonin in animals presenting as both out‐patients and in‐patients. In many cases, these animals also require sedation or general anesthesia while hospitalized for a variety of diagnostic or surgical procedures. Unfortunately, there is little evidence regarding the interactions of these drugs when they are combined with phenothiazines, benzodiazepines, α2‐adrenergic receptor agonists, injectable anesthetics, and inhalant anesthetics in dogs and cats. As a result, most decisions about sedative and anesthetic drug and dose selection in patients receiving gabapentin, trazodone, and melatonin are based on empirical observations of the animal’s temperament and demeanor in the immediate preanesthetic period. More information about gabapentin and trazodone is available in Chapter 25. In humans, phenothiazines have been used since the 1950s and were once the most widely used antipsychotic agents, prescribed to reduce symptoms in a wide variety of conditions including schizophrenia. They also have clinical applications for control of nausea and vomiting. Interestingly, while sedation is a well‐recognized side effect of phenothiazines in people, most antipsychotic drugs cause unpleasant subjective effects in nonpsychotic individuals [1]. Extrapyramidal side‐effects have been noted, typically with long‐term use in humans or overdose in veterinary patients (tremor, coma/catalepsy, and rigidity). Phenothiazines exhibit activity at a wide variety of receptor systems both centrally and peripherally, including adrenergic, muscarinic, dopaminergic, serotonergic, and histaminergic. The sedative effects of the phenothiazines are mediated primarily by blockade of dopamine receptors, specifically D2 receptors. This pre‐ and postsynaptic G‐protein‐coupled receptor blockade leads to a decrease in cyclic adenosine monophosphate (cAMP) and adenylate cyclase activity, calcium conductance, and alterations in postsynaptic potassium conductance. Blockade of central α1‐adrenergic, muscarinic, and histaminic (H1) receptors may also play a role in sedation. α1‐Adrenergic receptor antagonism mediates the decrease in blood pressure noted with this group of medications and may play a role in the decrease in thermoregulatory control (tendency toward poikilothermia), along with serotonin blockade. Acepromazine has been among the most widely used sedatives in veterinary practice for many years and is labeled for use in small and large animals in many countries. It is generally reliable as a sedative when given parentally, often at doses lower than the label dose, but failure to sedate and/or arousal with stimulation is not uncommon. Muscle relaxation with acepromazine is reasonably good, and the drug has been used to counteract muscle rigidly associated with other agents, such as ketamine. Acepromazine has no inherent analgesic properties when administered alone and is frequently given in combination with opioids to produce neuroleptanalgesia, a state characterized by both sedation and analgesia. There is no antagonist available to reverse the clinical anti‐dopaminergic effects of acepromazine. Acepromazine is highly protein‐bound with a large volume of distribution (Vd) [2]. Peak central nervous system (CNS) effects are usually noted 10–20 min after IV administration and 30–40 min after intramuscular (IM) administration [3]. It has a long duration of action with reported elimination half‐lives of 3–5 h in the horse [2,4] and 7 h in the dog [5]. In the horse, acepromazine is metabolized extensively in the liver with urinary excretion of metabolites [6]. In dogs, bioavailability after oral administration is approximately 20% [5]. The hemodynamic impact of acepromazine is variable, likely due to its diverse receptor interactions both centrally and peripherally. Acepromazine‐induced blockade of vascular α1‐adrenergic receptors results in peripheral vasodilation and has been reported to decrease stroke volume, cardiac output, and arterial blood pressure by 20–30% in conscious dogs administered 0.1 mg/kg IV [7,8]. More recently, hemodynamic effects were studied in conscious dogs administered IV acepromazine incrementally to cumulative doses of 0.01, 0.025, 0.05, and 0.1 mg/kg. Maximal decreases in stroke index and mean arterial pressure of 16% and 17%, respectively, were reported, but there were no significant changes in systemic vascular resistance or heart rate. The authors attributed the reduction in arterial pressure to reduced myocardial contractility [9]. Conscious horses administered 0.1 mg/kg IV showed a 20–30% decrease in mean aortic pressure and a 10–15% decrease in cardiac output [10]. Mean arterial pressure measured at the tail (not corrected for height) was significantly reduced in standing horses receiving 0.1 mg/kg acepromazine [11]. Standing horses receiving 0.04 mg/kg acepromazine IV had lower facial artery pressures but no real change in digital palmar arterial flow compared with saline‐treated horses [12]. Blood pressure can also be reduced in inhalant‐anesthetized dogs and horses. Dogs anesthetized with halothane receiving acepromazine at 0.05, 0.125, and 0.25 mg/kg IV displayed reductions in mean arterial pressure of 2.3%, 9.4%, and 16.8%, respectively, that were attenuated by phenylephrine [13]. Dogs anesthetized with propofol and isoflurane receiving IV acepromazine incrementally to cumulative doses of 0.01, 0.025, 0.05, and 0.1 mg/kg had increases in cardiac index but no significant changes in stroke index, systolic, diastolic, or mean arterial pressure, or heart rate [14]. Systemic vascular resistance index, however, decreased by 25% in the dogs receiving 0.05 and 0.1 mg/kg IV cumulative doses. Halothane‐anesthetized horses receiving IV acepromazine at 0.03 mg/kg showed a decrease in mean arterial blood pressure of up to 25% [15]. Clinical experience suggests that healthy animals premedicated with low, clinically applicable doses of acepromazine rarely display dramatic decreases in blood pressure during isoflurane or sevoflurane anesthesia; however, the drug’s hypotensive effects may be potentiated in hemodynamically unstable patients or in those receiving epidural or spinal local anesthetics. While changes in heart rate are variable and typically not significant, mild reflex tachycardia has been noted in response to decreased systemic vascular resistance and arterial blood pressure [7,10]. Premedication with acepromazine will increase the dose of epinephrine required to induce a ventricular arrhythmia, likely due to α‐adrenergic receptor blockade [16–18]. Acepromazine administration has little effect on pulmonary function. Respiratory rates often decrease, but arterial partial pressure of carbon dioxide (PaCO2) and pH are not typically affected because tidal volume is increased to maintain adequate minute ventilation [10,19]. Acepromazine has been shown to have no impact on respiratory rate and arterial blood gases in halothane‐anesthetized horses [15]. Pretreatment with acepromazine (0.035 mg/kg IM) in horses that were subsequently sedated or anesthetized with romifidine, butorphanol, and tiletamine–zolazepam reduced the impact of intrapulmonary shunt and ventilation–perfusion mismatching [20]. Acepromazine administration has a significant dose‐dependent impact on inhalant anesthetic requirements. In dogs, acepromazine at 0.2 mg/kg reduced halothane requirement by 28% and isoflurane requirement by 48% [21]. In another study of dogs anesthetized with halothane, IM administration of acepromazine at 0.02 and 0.2 mg/kg reduced the minimum alveolar concentration (MAC) by 34% and 44%, respectively [22]. In ponies receiving 0.05 mg/kg acepromazine IV, halothane MAC was reduced by 37% [23]. These across‐species MAC‐sparing effects indicate that patients receiving acepromazine must be closely monitored during anesthesia and the delivery of inhalant anesthetics adjusted accordingly. For many years, acepromazine administration was associated with seizure activity and was considered contraindicated in veterinary patients with seizure histories. This association dates back to a series of 1950’s case reports and experimental studies involving electroencephalographic (EEG) changes induced by another phenothiazine, chlorpromazine, in rabbits and people [24]. A subsequent 1970 study went on to report that high‐dose chlorpromazine altered EEG activity in epileptic dogs [25]. Since that time, however, several clinical studies have evaluated the effects of acepromazine in dogs with seizure histories [26,27], or undergoing procedures associated with increased seizure risk (e.g., myelography) [28], and found that acepromazine has no discernible effect on seizure frequency. Hematologic effects of acepromazine administration include decreased packed cell volume and potential effects on platelet aggregation. Hematocrit is reduced in some dogs and horses by 20–30% shortly after acepromazine administration as a result of splenic engorgement after α1‐adrenergic receptor blockade [2,3,12,29]. This effect can last several hours. Hemoglobin is similarly reduced by 14–22% in conscious dogs [9]. Evidence on the effects of acepromazine on platelet function in dogs is conflicting. One study demonstrated decreased platelet aggregation and altered coagulation times with acepromazine [30], while a subsequent thromboelastography study found no effect of acepromazine on platelet function [31]. Acepromazine has well‐recognized antiemetic properties that are attributed to antagonism of dopamine receptors in the chemoreceptor trigger zone. In dogs, administration of acepromazine 15 min prior to a variety of opioids reduced the incidence of vomiting from 45% to 18% [32]; however, its efficacy as an antiemetic was less than that of the neurokinin‐1 receptor antagonist, maropitant, prior to hydromorphone [33]. Lower esophageal sphincter tone and gastric emptying are reduced with acepromazine in dogs [34–36], but acepromazine with butorphanol was considered acceptable for restraint when performing upper gastrointestinal contrast studies in healthy dogs [36]. In horses, acepromazine administration is associated with similar effects on gastrointestinal motility [37,38]. In dogs, the combination of acepromazine–hydromorphone was less effective than dexmedetomidine–hydromorphone at reducing gastroesophageal reflux during anesthesia when compared to hydromorphone premedication alone [39]. Penile prolapse (priapism) in stallions has been associated with acepromazine administration [40,41]. A 2011 retrospective study by Driessen et al. showed that 2.4% of male horses developed penile prolapse lasting 1–4 h [41]. One stallion (0.02%) developed a prolapse lasting more than 12 h but less than 18 h. The incidence of permanent penile paralysis is extremely low but could be catastrophic in valuable breeding males. Glomerular filtration is maintained in dogs premedicated with acepromazine [42,43]. Acepromazine reduces urethral pressure in male cats anesthetized with halothane [44]. Acepromazine has antihistamine effects through its interactions at H1 receptors and should not be used for intradermal skin testing in dogs when wheal size and induration is assessed [45]. For more detailed information on the clinical use of acepromazine in the perianesthetic period in specific species, the reader is directed to species‐specific chapters elsewhere in this text. In healthy dogs and cats, acepromazine is commonly administered, often in combination with an opioid, for sedation or prior to induction of general anesthesia at doses ranging from 0.01–0.1 mg/kg IM, subcutaneous (SC), or IV (with the lower end of the dose range typically suggested for the IV route of administration). Lower doses have also been used during recovery from anesthesia to counteract emergence delirium or reduce hospital‐associated anxiety. In healthy dogs, the combination of acepromazine with an α2‐adrenergic receptor agonist (e.g., medetomidine and dexmedetomidine) prior to inhalant anesthesia has been investigated and, while effective, the combination does not appear to offer significant advantages [46–48]. There are anecdotal reports that dogs homozygous for the MDR1 (ABCB1) gene mutation, which results in non‐functional P‐glycoprotein, may show increased sensitivity to various sedative or analgesic drugs, including acepromazine. A small prospective study involving genotyped rough‐coated collies showed that those with the homozygous MDR1 mutation tended to have increased and prolonged sedation scores after 0.04 mg/kg acepromazine IV compared to heterozygous or normal dogs [49], but the clinical significance in this and other susceptible breeds remains to be determined. For more information on canine and feline P‐glycoprotein deficiency, the reader is referred elsewhere [50]. There have also been anecdotal reports of collapse and sudden death in Boxer dogs following acepromazine administration [51], though the underlying mechanism remains undefined. If this represents an enhanced vasovagal response, then use of a much‐reduced dose and/or pretreatment with an anticholinergic may preempt or blunt these adverse effects. In horses, acepromazine is administered during the pre‐ or postanesthetic periods, usually at doses ranging from 0.01–0.05 mg/kg IV. The use of acepromazine in the perianesthetic period has been associated with decreased risk of anesthetic morbidity and mortality [52]. An equine study found that acepromazine 0.02 mg/kg IV was similar to xylazine 0.3 mg/kg IV in its effects on unassisted recovery scores when administered after general anesthesia with isoflurane [53]. Acepromazine is used infrequently for sedation in pigs and, when administered alone, its sedative effects in this species are generally considered to be inadequate. In pigs, high doses of acepromazine (1.1 and 1.65 mg/kg IM) have been shown to reduce the incidence of halothane‐induced malignant hyperthermia in susceptible animals, while lower doses (0.55 mg/kg IM) only delay its onset [54]. Like the phenothiazines, butyrophenone derivatives (e.g., haloperidol) are considered first‐generation antipsychotics in humans and have been used for this purpose since the 1950s. In veterinary medicine, this class of drugs is rarely used for sedation in dogs, cats, horses, and domestic cattle; however, the butyrophenone azaperone continues to have clinical applications in swine, zoo animals, and wildlife. Azaperone’s mechanism of action, like acepromazine, involves antagonism of central dopaminergic (primarily D2) receptors. It also has a similar side effect profile, though with potentially less hypotension due to decreased affinity for vascular α1‐adrenergic receptors [1]. For more information on azaperone as a sedative in veterinary medicine, the reader is referred to species‐specific chapters (Chapters 55, 64, and 65) elsewhere in this text. The benzodiazepines are sedative‐hypnotic drugs that have been widely used in human and veterinary medicine for many years. The prototypical drug, diazepam (Valium®), was marketed in the 1960s for anxiety in people, and it became the top‐selling pharmaceutical in the United States throughout the 1970s and early 1980s [55]. While there are over 50 benzodiazepine agents used in people and animals for their behavior modifying and anticonvulsant properties [51], only diazepam, midazolam, and zolazepam will be discussed here as they have primary indications in the perianesthetic period. Benzodiazepines are considered controlled substances in many jurisdictions. In the United States, they are classified as Schedule IV drugs by the Drug Enforcement Administration. γ‐aminobutyric acid (GABA) is the primary inhibitory neurotransmitter in the CNS [56]. The GABAA receptor is a heteropentameric ligand‐gated (ionotropic) chloride channel assembled from a family of at least 19 subunits encoded by distinct genes, and these various subunit combinations give rise to numerous receptor subtypes [57]. Benzodiazepines augment GABA‐mediated inhibitory transmission at all levels of the CNS through positive allosteric modulation of the GABAA receptor, resulting in increased chloride conductance and hyperpolarization of postsynaptic cell membranes [58]. The benzodiazepine recognition site on the GABAA receptor is located at the interface between the α and γ2 subunits [59]. Barbiturates, ethanol, etomidate, and propofol also all modulate activity at GABAA receptors, and synergistic effects can result in significant CNS depression. As benzodiazepines enhance endogenous GABA binding to the receptor but lack direct agonist activity, these drugs are associated with a wide safety margin with regard to CNS depression. While all benzodiazepines share a common chemical structure characterized by a benzene ring linked to a seven‐membered diazepine ring, the response to benzodiazepines in vivo is multifactorial. Variations in receptor subunit configurations, their locations within the CNS, and a specific drug’s affinity for these receptors, as well as lipid solubility and other pharmacokinetic parameters, all impact the clinical effect of a particular drug and dose. In addition, the discovery of the benzodiazepine‐binding site on the GABAA receptor has long fueled speculation that the brain produces its own endogenous ligands for this site [60]. While a number of endogenous benzodiazepine ligands (“endozepines”) have been identified [61–63], one in particular (variably called “diazepam‐binding inhibitor” [DBI] and “acyl‐CoA‐binding protein” [ACBP]) has been shown to compete with diazepam for receptor occupation and may be involved in anxiety and mood disorders in humans [64]. Diazepam and midazolam are the most commonly used parenterally administered benzodiazepines in veterinary anesthesia, and their primary indications in the perianesthetic period include anxiolysis, sedation, and muscle relaxation. Their sedative effects, however, are species‐ and patient‐dependent and tend to be mild. In healthy dogs, cats, and horses, they may induce paradoxical behavioral effects including vocalization, excitement, and dysphoria, while in neonatal, geriatric, or systemically ill patients, their sedative effects may be more reliable [51]. Zolazepam is a benzodiazepine that is only available in combination with the dissociative agent, tiletamine, and marketed to veterinarians as Telazol® and Zoletil®. Zolazepam metabolism varies significantly across species, impacting both the duration of effect and quality of recovery with tiletamine–zolazepam combinations. More information about tiletamine–zolazepam can be found in Chapters 27, 55, and 65. Benzodiazepines do not affect nociceptive pathways and have no inherent analgesic properties. The effects of benzodiazepines are reversible with the specific antagonist, flumazenil. Diazepam is highly lipid‐soluble and is supplied for injection as a solution of an organic solvent including propylene glycol and ethanol. The formulation is viscous with a pH of 6.6–6.9 and may cause pain on injection. Uptake of diazepam after IM administration is erratic and unpredictable, and this route is not recommended [65]. Diazepam is light sensitive (i.e., undergoes photodegradation) and will bind to plastics if exposed to light for long periods [66,67]. In dogs, diazepam is rapidly absorbed from the gastrointestinal tract, with very high bioavailability, and is metabolized by hepatic microsomal enzymes via oxidative pathways [68,69]. In dogs, the elimination half‐life of IV diazepam has been reported to range between 14–16 min (0.5 mg/kg) [70] and 3.2 h (2 mg/kg) [71]. The authors of the former study suggested that this disparity was a result of their lower administered dose or inadequate assay sensitivity such that the terminal phase of their concentration–time curve was not detectable. In dogs given 0.5 mg/kg IV, the active metabolites nordiazepam (also known as “desmethyldiazepam”) and oxazepam appear rapidly and persist for longer than the parent compound, with elimination half‐lives of 2.2–2.8 and 3.5–5.1 h, respectively [70]. In Greyhounds receiving diazepam at 0.5 mg/kg IV, terminal half‐lives were 1.0 h for the parent drug, 2.4 h for nordiazepam, and 6.2 h for oxazepam, indicating that breed differences do exist [68]. Cats receiving very high IV doses of diazepam (5, 10, and 20 mg/kg) had an elimination half‐life of approximately 5.5 h. About 50% of the diazepam is rapidly metabolized to nordiazepam, which has a half‐life of 21 h in this species [72]. Cats have limited capacity to form glucuronide conjugates of diazepam and its metabolites, which can result in diazepam‐induced liver injury with repeated dosing in this species [73]. Horses administered 0.05–0.4 mg/kg diazepam IV demonstrated a half‐life of the parent drug ranging from 2.5–21.6 h. Nordiazepam and oxazepam were not detected in plasma but were present in urine, suggesting rapid excretion in this species [74]. Midazolam is the other benzodiazepine commonly administered to veterinary patients in the perianesthetic period. The drug has an open diazepine ring and is formulated at a pH of less than 4.0, which effectively stabilizes the diazepine ring and confers water solubility. On injection at physiologic pH, the diazepine ring closes, rendering the drug lipid‐soluble and able to cross the blood–brain barrier rapidly to produce its central effects [75]. This unique chemical structure, along with the fact that midazolam does not contain propylene glycol as a vehicle, means that it does not cause pain on IV injection and is reliably absorbed after IM administration across species. Like diazepam, midazolam is sensitive to light and undergoes photodegradation; it is also extensively metabolized by hepatic microsomal enzymes [76]. In dogs, the elimination half‐life of IV midazolam has been reported to range between 63 min (0.2 mg/kg) [77] and 77 min (0.5 mg/kg) [78]. Bioavailability following IM injection is reliable, ranging between 50% [77] and 90% [78]. Time to peak plasma concentration after IM administration of 0.2 or 0.5 mg/kg is 7.8 and 15 min, respectively [77,78]. Mucosal absorption after oral administration in dogs occurs in the oral cavity, esophagus, and stomach and is highly influenced by local pH [79]. The intranasal (IN) route of administration has been investigated in dogs, and both nasal drops and atomizer administration have been shown to be effective [80]. An IN gel formulation had a bioavailability of 70.4% in dogs [81]. Unlike diazepam, the rectal route of administration does not result in clinically useful plasma concentrations with midazolam, and this route is not recommended for acute seizure management [77]. Recent multicenter trials involving canine status epilepticus indicate that IN midazolam (administered via a mucosal atomizer) was superior to rectal diazepam [82] and comparable to IV midazolam for acute seizure management [83]; however, when the time required for IV catheter placement was taken into account, IN midazolam was considered the best option for controlling status epilepticus [83]. In cats administered midazolam at 0.3 mg/kg IV under sevoflurane anesthesia, the elimination half‐life was 79 min, and peak plasma concentrations of the active metabolite 1‐hydroxymidazolam were reached at 15 min after midazolam administration [84]. This study also reported high inter‐individual variability in plasma concentrations of 1‐hydroxymidazolam, which had a low clearance and persisted for longer compared to the parent drug. The combination of IN midazolam and ketamine has been evaluated in cats, and this route was associated with a similar onset, degree, and duration of sedation compared to IM administration and was well‐tolerated by the cats [85]. In horses, the elimination half‐life of IV midazolam has been reported to be 216 min (0.05 mg/kg) and 408 min (0.1 mg/kg), demonstrating dose‐dependent kinetics [86]. Benzodiazepines induce relatively minor cardiovascular changes across species. In healthy dogs, clinically relevant IV doses of diazepam and midazolam have minimal effects on cardiac output, stroke volume, systemic vascular resistance, and coronary arterial blood flow [87]. Similarly, in horses, heart rate, cardiac output, and mean pulmonary arterial, aortic, and right atrial pressures are unchanged after IV diazepam (0.05–0.4 mg/kg) [74]. Consequently, these drugs are commonly suggested for veterinary patients with cardiovascular disease [88,89]. In dogs, however, there is no evidence suggesting that the combination of a benzodiazepine with an induction agent (e.g., propofol or alfaxalone) improves cardiovascular function compared to the induction agent alone. The effects of benzodiazepines on pulmonary function are also minimal. Arterial blood gas values are unchanged in horses receiving IV diazepam (0.05–0.4 mg/kg) [74], and in swine receiving IV midazolam (0.1–1.0 mg/kg) [90]. At high doses, when combined with other CNS depressants, or when administered to patients with significant pulmonary disease, benzodiazepine‐induced respiratory depression may be evident. Diazepam and midazolam modestly reduce injectable and inhalant anesthetic requirements when administered in the perianesthetic period. In healthy dogs, both diazepam and midazolam have been shown to reduce the induction dose requirements for propofol and alfaxalone, but the addition of the benzodiazepine does not necessarily improve cardiopulmonary stability over propofol or alfaxalone alone [91–94]. Inhalant‐sparing effects have also been documented with diazepam (0.044 mg/kg IV) reducing halothane MAC by 29% in ponies [95] and the combination of diazepam‐fentanyl reducing isoflurane MAC by 74% compared to 54% with fentanyl alone in dogs [96]. In isoflurane‐anesthetized dogs, IV midazolam (loading dose and constant‐rate infusion [CRI]) dose‐dependently reduces MAC, but a ceiling effect is evident with maximal MAC reduction of approximately 30% [97]. Benzodiazepines produce muscle relaxation through inhibitory effects on polysynaptic reflexes and spinal internuncial transmission and, at very high doses, may depress skeletal neuromuscular junction transmission [98]. At clinical doses, they may cause muscle weakness and ataxia and, in some species, this may progress to recumbency. Benzodiazepines are frequently co‐administered with dissociative agents (ketamine and tiletamine) to counteract muscle rigidity associated with these drugs. Benzodiazepines have well‐recognized anticonvulsant properties and are able to inhibit the development and spread of epileptiform electrical activity in the CNS [98]. In the case of diazepam, its active metabolites, nordiazepam and oxazepam, are associated with 25–33% less anticonvulsant activity than the parent drug [99]. They generally have a favorable profile with regard to cerebral perfusion, reducing cerebral oxygen consumption to a greater extent than cerebral blood flow [100]. In dogs and cats, diazepam and midazolam are commonly administered in the preanesthetic period, often in combination with opioids, or as co‐induction agents with ketamine, propofol, or alfaxalone. In hemodynamically unstable small animal patients, the combination of a benzodiazepine and full μ‐opioid receptor agonist may be used to induce general anesthesia. While midazolam is considered more potent than diazepam in humans, dose ranges in dogs and cats are similar (0.1–0.4 mg/kg IV), and the two drugs are often used interchangeably in the perianesthetic period. Anticonvulsant doses for both drugs tend to be higher (0.5–1.0 mg/kg IV). As discussed above, the IM route of administration is not recommended for diazepam due to pain and erratic absorption associated with the propylene glycol vehicle. The availability of multiple effective routes of administration for midazolam make it a more versatile option in the clinical setting. In general, the sedative effects of benzodiazepines in healthy dogs and cats are minimal and unreliable if not combined with other sedative‐hypnotic agents. Paradoxical excitation characterized by restlessness, agitation, and vocalization is common as doses increase, especially in cats [78,101]. Behavioral disinhibition due to anxiolysis may even provoke aggressive behavior in some animals. In adult horses, midazolam (0.5–0.1 mg/kg IV) alone produces minimal sedation but is associated with ataxia, weakness, postural sway, and agitation [86], making it unsuitable for procedures requiring standing sedation. In neonatal foals, however, the combination of an IV benzodiazepine with an opioid tends to produce reliable sedation but may be accompanied by weakness leading to recumbency. For induction of general anesthesia in adult horses sedated with an α2‐adrenergic receptor agonist, either diazepam or midazolam (0.05–0.1 mg/kg IV) is commonly co‐administered with ketamine to enhance muscle relaxation, smooth induction, and facilitate endotracheal intubation. With commercial preparations of guaifenesin no longer available in many countries, midazolam has also been investigated as an alternative to guaifenesin for equine total intravenous anesthesia (TIVA). The infusion of midazolam–xylazine–ketamine after induction appears to be suitable for extending the duration of anesthesia for up to 1 h in horses [102]. In addition to dogs, cats, and horses, midazolam has been used in a wide variety of species including swine, goats, sheep, alpacas, ferrets, rabbits, guinea pigs, and birds [103–108]. In general, small ruminants, ferrets, rabbits, and birds tend to sedate reasonably well with benzodiazepines, often in combination with other drugs such as opioids, α2‐adrenergic receptor agonists, alfaxalone, and ketamine. The IN route of administration has been studied in rabbits [107] and various avian species [109,110]. Flumazenil is a competitive antagonist with a high affinity for the benzodiazepine recognition site on the GABAA receptor but no appreciable agonist activity at the site. As the drug is specific to the benzodiazepine‐binding site on the receptor, it does not antagonize the CNS effects of other anesthetic agents interacting with the GABAA receptor such as propofol, alfaxalone, etomidate, and barbiturates. Flumazenil attenuates the CNS depressant effects of benzodiazepines and has been shown to reverse their EEG changes in dogs [111,112]. When administered in the absence of a benzodiazepine, the drug does not appear to induce appreciable CNS stimulation (e.g., agitation, excitement, and tachypnea) in veterinary species [51]. While flumazenil is thought to antagonize the effects of endogenous benzodiazepine‐like substances (endozepines), which are elevated in human patients with hepatic encephalopathy, there is little evidence supporting its use for clinical management of hepatic encephalopathy in people [113]. As a conventional antagonist (as opposed to an inverse agonist that acts as a negative allosteric modulator of GABA receptor function), flumazenil appears devoid of direct cardiovascular, respiratory, or musculoskeletal effects but is effective in reversing benzodiazepine‐induced changes in these systems. In humans, however, antagonism of benzodiazepine‐induced respiratory depression may be less predictable [98]. There is little pharmacokinetic information available about flumazenil in veterinary species. Reversal of excessive benzodiazepine‐induced sedation in the perianesthetic period may be accomplished in dogs with IV doses of 0.01–0.04 mg/kg, and in horses with IV doses of 0.01–0.02 mg/kg. Repeated doses may be necessary if sedation persists or if re‐sedation is noted. In humans, it is well recognized that flumazenil may precipitate abstinence syndrome in individuals that have developed physiologic dependence to chronic oral benzodiazepines. This phenomenon has been reported experimentally in dogs, though the doses of flumazenil studied were very high [114]. Furthermore, flumazenil may precipitate seizures and cardiac arrhythmias in humans that have ingested an overdose of benzodiazepines with tricyclic antidepressants. An experimental study involving dogs administered infusions of midazolam and amitriptyline that were suddenly reversed with flumazenil demonstrated the potential for convulsions and arrhythmias in this species [115]. For these reasons, flumazenil is not routinely recommended for the treatment of benzodiazepine or mixed drug overdose in humans but should be reserved for selected patients where the benefits of flumazenil administration outweigh the potential risks [116]. Xylazine was discovered in 1962 as an antihypertensive agent by Farbenfabriken Bayer AG in Leverkusen, Germany, many years before its mechanism of action as an α2‐adrenergic receptor agonist was elucidated [117]. During early clinical trials in people, xylazine induced excessive CNS depression, and the drug was abandoned for human use and introduced to the veterinary market as a sedative, analgesic, and muscle relaxant [117]. Early reports of its use in animals began appearing in the late 1960s and early 1970s [118–122], and it soon became one of the most commonly administered sedatives in veterinary medicine, especially in large animals. It was not until 1981 that xylazine’s CNS depressant effects were definitively attributed to its actions at central α2‐adrenergic receptors [123]. Over the next 40 years, that discovery would spearhead development of new, more specific veterinary α2‐adrenergic receptor agonists including detomidine, romifidine, and medetomidine/dexmedetomidine. Dexmedetomidine is also used in human patients for procedural sedation, ICU sedation in mechanically ventilated patients, and as an adjunct to general anesthesia. While α2‐adrenergic receptor agonists have not historically been classified as controlled substances, concerns about illicit xylazine use as an emerging public health threat, coupled with the potential for diversion from veterinary channels, is leading to regulatory changes involving xylazine in several jurisdictions including in the United States and Canada. The clinically desired effects of these drugs (sedation, analgesia, and muscle relaxation) are a result of their interactions with α2‐adrenergic receptors located both pre‐ and postsynaptically in the CNS. In addition, α2‐adrenergic receptors are also widely distributed throughout peripheral tissues with pre‐ and postsynaptic receptors found in many organs and postsynaptic (extrajunctional) receptors located on vascular endothelium and on platelets. These peripheral receptors mediate many of the adverse effects associated with α2‐adrenergic receptor agonist administration in the clinical setting. The α2‐adrenergic receptor is a G‐protein‐coupled receptor that has been recognized as four distinct subtypes: α2A, α2B, α2C, and α2D. The α2A subtype was first discovered in human platelets and rabbit spleen, the α2B subtype in rat lung and kidney, the α2C subtype in opossum kidney, and the α2D subtype in rat submaxillary gland and bovine pineal gland [124]. Because the α2A and α2D subtypes are structurally similar in mammalian species, they are now believed to be the same receptor but with differing pharmacologic profiles in humans, dogs, rabbits, pigs, and chickens (α2A) versus rats, mice, and cattle (α2D) [125,126]. To further complicate the nomenclature, a fourth non‐mammalian receptor subtype, also called “α2D,” has also been identified in zebrafish [127]. Despite the potential for subtype‐specific effects, the distinction is not clinically relevant as none of the currently available α2‐adrenergic receptor agonists or antagonists is subtype specific. Agonist binding at all subtypes by endogenous ligands (norepinephrine, epinephrine) or exogenous α2‐adrenergic receptor agonists results in allosteric inhibition through Gi/o leading to inhibition of adenylate cyclase activity and decreased cAMP concentrations. Subsequent inhibition of Ca2+ entry and activation of outward‐opening K+ channels result in membrane hyperpolarization and suppression of neuronal firing [128,129]. While inhibition of adenylate cyclase is considered the classic α2‐adrenergic receptor signaling pathway, other effector mechanisms also appear to play a role. The α2A and α2C subtypes predominate in the CNS and are largely responsible for the sedative, analgesic, and sympatholytic effects of α2‐adrenergic receptor agonists [130]. The locus coeruleus in the medial dorsal pons is the major noradrenergic nucleus of the brain and contains dense populations of α2‐adrenergic receptors. Activation of presynaptic receptors here results in inhibition of norepinephrine release and reduced activity in ascending noradrenergic pathways, resulting in hypnosis and sedation [131,132]. Activation of this negative feedback loop may also produce reductions in heart rate and blood pressure and attenuation of the sympathetic stress response. At the level of the spinal cord dorsal horn, stimulation of α2‐adrenergic receptors inhibits nociceptive neurons and reduces the release of norepinephrine and substance P. Although there is some evidence for supraspinal (i.e., descending modulation via the locus coeruleus) and peripheral sites of analgesic action, the spinal mechanism is thought to be responsible for most of the analgesia associated with α2‐adrenergic receptor agonists [132]. The α2B subtype is located primarily on vascular smooth muscle and largely mediates vasoconstriction [132]. Some effects of α2‐adrenergic receptor stimulation do not appear to be mediated through G‐protein coupling, such as inhibition of platelet aggregation. In addition to their effects at α2‐adrenergic receptors, all α2‐adrenergic receptor agonists currently in clinical use also have some impact on α1‐adrenergic receptors and most also activate nonadrenergic imidazoline receptors (Table 22.1). Activation of vascular α1‐adrenergic receptors contributes to vasoconstriction and increased systemic vascular resistance, while activation of central α1‐adrenergic receptors has been associated with increased arousal, restlessness, vigilance, and locomotor activity in animals [133]. In theory, α2‐adrenergic receptor agonists that are less selective for α2‐adrenergic receptors may be more likely to cause adverse effects such as paradoxical excitement and locomotor activity. Of the commonly used α2‐adrenergic receptor agonists, xylazine has the lowest α2:α1 adrenergic receptor binding ratio (Table 22.1). Table 22.1 α2:α1 adrenergic receptor selectivity and imidazoline activity for selected α2‐adrenergic receptor agonists and antagonists. All of the α2‐adrenergic receptor agonists currently used in veterinary medicine, except for xylazine, have structural similarities to imidazoline and thus may also activate the various subtypes of imidazoline receptors (Table 22.1). Differentiating α2‐adrenergic versus imidazoline receptor‐mediated effects is difficult and there appears to be considerable overlap. The I1 receptor plays a role in cardiovascular regulation and has been implicated in modulation of sodium excretion and urine output, inhibition of catecholamine‐induced arrhythmias, and possibly neuroprotective effects [134]. The I2 receptor may be involved in control of central noradrenergic and hypothalamic–pituitary–adrenal axis activity, regulation of intestinal motility, and opioid‐induced antinociception [134]. The I3 receptor may play a role in insulin secretion and contribute to α2‐adrenergic agonist‐induced hyperglycemia [135]. The α2‐adrenergic receptor agonists are used extensively in veterinary patients to provide chemical restraint, sedation, analgesia, and as adjuncts to general anesthesia. They are a versatile class of drugs and can be administered via a variety of routes including IV, IM, IV CRI, oral transmucosal (OTM), IN, neuraxial (epidural, spinal), and perineural. In some species, notably cats, α2‐adrenergic receptor agonists reliably induce vomiting and may be used as emetics. In general, the onset of action of α2‐adrenergic receptor agonists after IV administration is rapid (within minutes), and peak effects are noted shortly thereafter. IM administration of α2‐adrenergic receptor agonists is typically associated with rapid absorption, but plasma concentrations show significant variation in the first 60 min following IM administration across species and between individuals of the same species. Differences in study design and pharmacokinetic methodology can further confound interpretation. While the SC route of administration has not historically been recommended due to concerns about poor absorption related to peripheral vasoconstriction, there is some evidence in humans [139,140] and horses [141] to suggest that the SC route may be effective and could be clinically useful. Other alternate routes of systemic administration such as OTM and IN have also been investigated for specific drugs. As the α2‐adrenergic receptor agonists are highly lipophilic, neuraxial (epidural, spinal) administration to enhance and prolong local anesthetic‐induced regional anesthesia results in significant systemic absorption and is usually accompanied by dose‐dependent CNS depression (sedation) and cardiovascular effects that are comparable to systemic α2‐adrenergic receptor agonist administration. Perineural administration to enhance and prolong local anesthetic‐induced peripheral nerve blockade tends to be associated with lower plasma drug concentrations and possibly fewer systemic side effects. For xylazine, pharmacokinetic parameters have been described in dogs, horses, cattle, and sheep by a two‐compartment model [142]. In the same study, elimination half‐lives after IV administration of varying species‐specific doses ranged between 22 min in sheep and 50 min in horses. After IM administration, peak plasma concentrations were attained within 12–14 min in all species, but mean bioavailability was higher in dogs (74%) compared to horses (45%) and sheep (41%) [142]. In racing horses, the pharmacokinetics of xylazine have been re‐evaluated more recently from a regulatory medicine perspective. After IV administration of 0.3–0.4 mg/kg xylazine, drug disposition was found to fit a three‐compartment model with reported systemic clearances of 12.7 and 15.8 mL/min/kg, β half‐lives of 2.79 and 3.53 h, and γ half‐lives of 26 and 29.8 h [143,144]. Identification of this third compartment, and the associated prolonged γ half‐lives, reflects increased sensitivity of the analytical methods used and may not be clinically relevant in the perianesthetic period. In conscious horses receiving xylazine at 0.5 mg/kg IV followed by a CRI of 1 mg/kg/h for 2, 4, and 6 h, plasma concentration–time curves were best described by a one‐compartment model, and xylazine did not appear to accumulate in plasma or tissues with longer infusion periods [145]. In horses recovering from isoflurane anesthesia, a single IV dose of 0.2 mg/kg xylazine was associated with significantly slower plasma kinetics compared to a single IV dose of 0.875 μg/kg dexmedetomidine, but no differences in time to stand or recovery quality were observed [146]. The intraosseous route has also been shown to be effective for administration of xylazine in horses [147]. Regarding detomidine, pharmacokinetic studies in horses at IV doses ranging between 10 and 80 μg/kg suggest a two‐compartment model with some evidence of dose‐dependent elimination [148–150]. In resting horses, 40 μg/kg IV had a median half‐life of 26 min, a volume of distribution of 585 mL/kg, and a median clearance of 15 mL/min/kg. After supramaximal exercise, these parameters increased to 46 min, 1296 mL/kg, and 20 min, respectively [150]. In another equine study, the mean elimination half‐life of a 30 μg/kg dose was 26 min for the IV route of administration versus 53 min for the IM route [151]. In cattle, 80 μg/kg of detomidine IV or IM showed rapid absorption and distribution, with an elimination half‐life of 1.3 h following the IV dose and 2.6 h following the IM dose [148]. Injectable detomidine has also been evaluated for OTM administration, and an oromucosal gel is marketed in some countries for use in horses. In horses, the mean bioavailability for the gel was 22% after sublingual administration of 40 μg/kg, compared with 38.2% following the same dose of injectable detomidine administered IM [152]. In addition to horses, OTM detomidine administration has been evaluated in other species including calves [153], dogs [154–156], ferrets [157], rabbits [158], and cats [159] with varying results. Though bioavailability with the OTM route of administration was lower, clinically relevant sedation was achieved in dogs, ferrets, and cats. In horses and alpacas, detomidine gel administered intravaginally resulted in clinically relevant sedation and bioavailabilities of 25% and 20%, respectively [160,161]. Detomidine has also been administered intranasally via a mucosal atomizer in sheep [162]. Romifidine administered at 80 μg/kg IV to horses resulted in plasma concentrations fitted to a two‐compartment model with a prolonged elimination half‐life of 135 min [163]. Administration of a lower dose (10 μg/kg IV) prior to exercise in horses confirmed the two‐compartment model and the extended elimination half‐life [164]. The pharmacokinetics of dexmedetomidine and racemic medetomidine (a mixture of both dexmedetomidine and levomedetomidine) are very similar in dogs [165]. All of the pharmacologic actions of medetomidine are due to the dextrorotatory isomer, which is isolated in dexmedetomidine. Administration of medetomidine at 40 μg/kg IV and dexmedetomidine at 20 and 10 μg/kg IV produced peak sedation at 10–20 min at plasma concentrations of 18.5, 14, and 5.5 ng/mL, respectively, in dogs. Terminal half‐lives of 0.96, 0.78, and 0.66 h were reported [166]. Administration of levomedetomidine produced no apparent sedation or analgesia. Clearance of levomedetomidine was faster [166]. The pharmacokinetics of IM dexmedetomidine (10 μg/kg) administered to healthy dogs have been reported and show significant variations in individual plasma concentrations. In cats anesthetized with isoflurane and administered 10 μg/kg dexmedetomidine IV over 5 min, a mean terminal half‐life of 198 min was reported [167]. In horses and ponies, IV dexmedetomidine or medetomidine results in rapid reductions in plasma drug concentrations with reported elimination half‐lives ranging between 8 and 29 min depending on the study [168–170]. In horses recovering from isoflurane anesthesia, a single IV dose of 0.875 μg/kg dexmedetomidine was associated with significantly faster plasma kinetics compared to a single IV dose of 0.2 mg/kg xylazine, but no differences in time to stand or recovery quality were observed [146]. Similar to detomidine, OTM administration of injectable medetomidine and dexmedetomidine has been investigated in cats and dogs, and an oromucosal dexmedetomidine gel is also marketed for noise phobia in dogs in a number of countries. In cats, OTM medetomidine produced similar sedation compared to IM injection but took longer to achieve peak plasma concentrations (43 versus 21 min). Individual variation was suspected to be secondary to salivation and drug loss [171]. Dexmedetomidine has also been administered to cats using the OTM route, sometimes combined with buprenorphine [172–174]. In dogs, 20 μg/kg of dexmedetomidine administered OTM was compared to a 5 μg/kg IV dose. Despite a bioavailability of only 11.2%, OTM dexmedetomidine produced a similar degree of sedation and a prolonged duration of action compared to IV administration [175]. The OTM route has also been evaluated for a combination of dexmedetomidine and methadone in dogs [176]. IN dexmedetomidine has been evaluated for sedation in children and, more recently, in dogs and cats. In healthy Beagles, 20 μg/kg dexmedetomidine administered IN resulted in slower absorption and lower plasma concentrations at all time points compared to IM administration, despite clinically similar sedation effects [177]. Perineural administration of 0.5 μg/kg dexmedetomidine with levobupivacaine for inferior alveolar and infraorbital nerve blocks in anesthetized dogs resulted in lower plasma dexmedetomidine concentrations compared to IV administration [178]. In most species, dexmedetomidine appears to undergo hepatic metabolism to pharmacologically inactive metabolites by hydroxylation, oxidation, or conjugation. In human intensive care unit patients, where the drug is commonly used as a sedative, high inter‐individual pharmacokinetic variability has been described with factors such as body size, hepatic impairment, plasma albumin, and cardiac output reported to influence pharmacokinetics. The α2‐adrenergic receptor agonists in current clinical use induce significant cardiovascular effects that are typically described as biphasic. Initially, activation of postsynaptic α1‐ and α2B‐adrenergic receptors results in peripheral vasoconstriction, increased systemic vascular resistance, and increased arterial blood pressure. These peripheral changes induce a baroreceptor‐mediated reflex bradycardia, which, while it may attenuate hypertension to some extent, results in significant reductions in cardiac output. While there is substantial variation depending on the drug, the dose, the route of administration, and the species, initial hemodynamic changes typically include bradycardia, with or without bradyarrhythmias, and hypertension. At the same time, activation of central presynaptic α2A‐ and α2C‐adrenergic receptors results in reduced norepinephrine release and sympathetic outflow, which perpetuates bradycardia and the reduction in cardiac output. The net result of these secondary hemodynamic changes is usually bradycardia and hypotension. At clinically relevant doses, α2‐adrenergic receptor agonists have been shown to decrease cardiac output in most species by greater than 50% [179–182]. This reduction in cardiac output is largely due to changes in cardiac loading conditions (increased systemic vascular resistance and bradycardia) rather than direct effects on myocardial contractility [183,184]. While these effects are often considered to be dose‐dependent, even low bolus doses may induce adverse cardiovascular effects [179], and there seems to be a “ceiling effect” whereby higher doses do not necessarily worsen cardiovascular parameters [166]. It is important to note that the influence of other drugs administered concurrently (e.g., inhalant anesthetics) and the route of administration (e.g., IV bolus, versus IM, versus low‐dose CRI) will also impact the magnitude of cardiovascular changes [185,186]. The reduction in cardiac output is not uniform across all tissue beds with blood flow redistributed away from nonessential regions such as skin, adipose tissue, skeletal muscle, and intestine, and is redirected to essential organs such as brain, heart, and kidneys [179,187]. α2‐Adrenergic receptor agonists do not adversely impact myocardial perfusion and may beneficially modulate coronary blood flow during myocardial ischemia [188,189]. The bradycardia induced by α2‐adrenergic receptor agonists can be significant and, in addition to sinus bradycardia, vagally mediated bradyarrhythmias such as first‐ and second‐degree atrioventricular block are commonly observed in some species (dogs and horses) [190]. More serious bradyarrhythmias such as third‐degree atrioventricular block and sinus arrest are rare. The less selective α2‐adrenergic receptor agonist, xylazine, has been shown to reduce the arrhythmogenic dose of epinephrine (ADE) in halothane‐ and isoflurane‐anesthetized dogs [191,192]; however, the more selective agents (with less α1‐adrenergic receptor activity), such as detomidine, medetomidine, and dexmedetomidine, do not alter the ADE and may even have a protective effect against ventricular arrhythmias [193–195]. Routine administration of an anticholinergic to prevent or counteract bradycardia during the initial phase of α2‐adrenergic receptor agonist‐mediated peripheral vasoconstriction is not recommended. While anticholinergic administration in the face of increased systemic vascular resistance may increase heart rate, cardiac output does not increase proportionally and other adverse effects have been documented including hypertension, increased myocardial oxygen consumption, and arrhythmias [190,196–198]. Once the initial increase in systemic vascular resistance begins to wane leaving the central sympatholytic effects to predominate, anticholinergic administration may be considered on a case‐by‐case basis to treat persistent bradycardia, especially if it is accompanied by hypotension. α2‐Adrenergic receptor agonists induce centrally mediated reductions in both respiratory rate and minute ventilation [199,200]; however, increases in arterial carbon dioxide tension (PaCO2) and decreases in arterial oxygen tension (PaO2) are not usually significant in most species [181,199–202]. Co‐administration of α2‐adrenergic receptor agonists with other sedatives, opioids, or anesthetics may lead to more significant impairment of ventilatory drive predisposing to hypercapnia and possibly hypoxemia. In isoflurane‐anesthetized mechanically ventilated dogs, however, a low‐dose dexmedetomidine CRI (1 μg/kg/h IV), with or without a 1 μg/kg IV loading dose, actually improved oxygenation and respiratory system compliance, and reduced intrapulmonary shunt fraction and airway resistance [203]. In humans, dexmedetomidine is commonly used for sedation in patients requiring mechanical ventilation in intensive care settings. Administration of α2‐adrenergic receptor agonists commonly causes mucous membranes to appear “muddy” or pale blue/gray as peripheral vasoconstriction slows blood flow in the periphery. The prolonged capillary transit time allows greater oxygen extraction, resulting in a greater amount of deoxygenated hemoglobin being present at the venous end of the capillary. This often exceeds the 5 mg/dL threshold of deoxygenated hemoglobin associated with the clinical observation of cyanosis. It should be appreciated that PaO2 values in this situation are often normal or near normal; thus, the observation of cyanosis in this context has a different implication than when it is noted in patients with arterial hypoxemia due to pulmonary disease or severe hypoventilation. Sheep are uniquely susceptible to hypoxemia following administration of α2‐adrenergic receptor agonists due to activation of pulmonary intravascular macrophages, which damage capillary endothelium and alveolar type I cells, leading to intra‐alveolar hemorrhage and interstitial and pulmonary edema [204,205]. α2‐Adrenergic receptor agonist‐mediated sedation results from activation of α2‐adrenergic receptors in the locus coeruleus of the pons and the rostroventral lateral medulla (the primary areas for sympathetic outflow from the CNS). This reduces norepinephrine release, which diminishes arousal as the discharge frequency of tracts into the cortex slows. Rigidity, seizures, and/or excitement have been noted following accidental intracarotid injection of xylazine in horses. Failure to sedate, or paradoxical excitement, can result when animals are excited, fearful, in pain, or otherwise stressed. These conditions result in high circulating catecholamine levels, which are difficult to overcome with α2‐adrenergic receptor agonist administration [190,206]. Animals that appear sedated may be suddenly aroused and display aggressive behavior if disturbed and may demonstrate increased sensitivity to sound and tactile stimulation [206]. Activation of central α1‐adrenergic receptors has been shown to functionally antagonize the hypnotic response to α2‐adrenergic receptor agonists [207], and α1‐adrenergic stimulation may contribute to some of these paradoxical behaviors, especially with less selective agents such as xylazine. Analgesia results from agonist binding to pre‐ and postsynaptic α2‐adrenergic receptors at various points in nociceptive pathways including the substantia gelatinosa of the spinal cord dorsal horn and the locus coeruleus of the brainstem [208]. Afferent input into the dorsal horn from peripheral nociceptors continues but is modulated and dampened by decreases in norepinephrine and substance P release resulting from presynaptic α2‐adrenergic receptor binding. Postsynaptic α2‐adrenergic receptor binding in the dorsal horn and supraspinally results in neuronal hyperpolarization and inhibition of nociceptive neurons. Interaction with imidazoline receptors (specifically the I2 receptor) may also play a role in α2‐adrenergic receptor agonist analgesia through modulation of opioid antinociception [134]. It has been noted that analgesic effects seem to be shorter‐lived than sedative effects in a number of species, and plasma drug concentrations exceeding those associated with sedation may be required to produce antinociception [149]. While α2‐adrenergic receptors and opioid receptors share similar molecular machinery and are present in similar locations throughout the CNS, α2‐adrenergic receptor agonists and opioids have sufficiently different mechanisms of analgesia to result in synergism when co‐administered. Co‐administration with other analgesics such as local anesthetics and ketamine via a variety of routes (systemically, neuraxially, or in peripheral nerve blocks) is common in clinical practice. In humans, dexmedetomidine has been shown to significantly improve postoperative outcomes when used as part of an Enhanced Recovery After Surgery (ERAS) protocol and is recommended as part of a multimodal opioid‐sparing approach [209]. The central effects of α2‐adrenergic receptor agonists (both sedative and analgesic) induce significant anesthetic‐sparing effects when combined with injectable or inhalant anesthetics. Effects are dose‐dependent and vary by drug, species, and route of administration, but α2‐adrenergic receptor agonist‐induced reductions in anesthetic requirements of up to 90% have been reported [51,190]. This underscores the importance of titrating injectable and inhalant anesthetic doses to effect when combined with this potent class of drugs. The effects of α2‐adrenergic receptor agonists on cerebral hemodynamics and intracranial pressure (ICP) have been studied experimentally in animals and clinically in humans with various types of neurologic disease. In isoflurane‐anesthetized dogs, medetomidine did not change ICP [210], and dexmedetomidine reduced cerebral blood flow with no alteration in cerebral metabolic rate of oxygen [211]. In humans, these neuroprotective effects (i.e., reduced cerebral blood flow and ICP) have led to dexmedetomidine’s use in neurosurgical patients and those with traumatic brain injury to provide sedation and lower opioid requirements [212]. In veterinary patients with neurologic disease, the decision to include an α2‐adrenergic receptor agonist in the anesthetic plan should be made on a case‐by‐case basis after considering the potential for respiratory depression when these drugs are combined with opioids and anesthetic agents, the significant hemodynamic effects, the potential for hyperglycemia, and the potential to induce vomiting and elevate ICP. Thermoregulation is impaired in patients administered α2‐adrenergic receptor agonists due to CNS depression and reduced muscle activity. However, as these drugs cause peripheral vasoconstriction and redistribution of blood flow from the skin to the core, hypothermia may be less significant compared to drugs that cause peripheral vasodilation, such as acepromazine and inhalant anesthetics. Regardless, body temperature should be monitored, especially in small animals, and thermoregulatory support provided as needed. The effects of α2‐adrenergic receptor agonists on pupil size vary by species with mydriasis reported in cats, rats, and rabbits, and either no change in pupil size or miosis reported in dogs. In most species, reductions in intraocular pressure have been reported, but this effect is less consistent in dogs. The potential for α2‐adrenergic receptor agonist‐induced nausea and vomiting must be considered when planning to sedate or anesthetize small animal patients that may be adversely affected by fluctuations in intraocular pressure [190]. α2‐Adrenergic receptor agonists induce nausea and vomiting in dogs and cats through stimulation of the chemoreceptor trigger zone, which is in close proximity to the locus coeruleus [213–215]. Depending on the drug, dose, route of administration, and other drugs administered concurrently, α2‐adrenergic receptor agonists may induce vomiting in up to 90% of cats and 30% of dogs [51,190]. Gastrointestinal transit time is prolonged and gastric acid secretion, reticuloruminal contractions, and colonic motility are reduced with α2‐adrenergic receptor agonists [216–218]. These effects are reversible with α2‐adrenergic receptor antagonists confirming that effects on gastrointestinal motility are mediated by α2‐adrenergic receptors. The diuretic effects of α2‐adrenergic receptor agonists are mediated through multiple mechanisms including reduced production or release of antidiuretic hormone (ADH) from the pituitary gland; inhibition of ADH activity on renal collecting tubules; and enhanced sodium excretion [219–222]. The renin–angiotensin–aldosterone system is also impacted directly through activation of renal α2‐adrenergic receptors and indirectly through α2‐adrenergic receptor agonist‐induced hypertension, resulting in reduced renin secretion and enhanced diuresis [223]. α2‐Adrenergic receptor agonists also impact micturition resulting in decreased micturition pressure, urinary bladder capacity, micturition volume, and residual volume, and these effects appear to be mediated largely through central (spinal) mechanisms [224]. Clinically, the net result of these effects is that animals administered α2‐adrenergic receptor agonists tend to produce large volumes of dilute urine. Myometrial tone and intrauterine pressure can increase with α2‐adrenergic receptor stimulation [225–229]; however, the clinical effects of α2‐adrenergic receptor agonist administration are not consistent, and there is considerable variation with different drugs, doses, routes of administration, timing within the reproductive cycle, and species. Classically, xylazine was reported to induce oxytocin‐like contraction of the bovine uterus [225] and, based on anecdotal reports of premature labor and spontaneous abortions in heifers, its use in heavily pregnant cattle was cautioned despite minimal evidence implicating xylazine in these cases. Regarding the more specific α2‐adrenergic receptor agonists, detomidine (< 60 μg/kg IM in the cow and horse) [230,231] and medetomidine (< 20 μg/kg IV in the dog) [229] have been shown to decrease myometrial contractions. However, higher doses of medetomidine (40–60 μg/kg IV) did increase electrical activity of the pregnant canine uterus [229]. Fluctuations in circulating levels of steroid hormones throughout pregnancy may impact these changes with estrogen upregulating excitatory α‐adrenergic receptors and progesterone upregulating inhibitory β‐adrenergic receptors in the reproductive tract [229,232]. Overall, these reproductive effects, combined with potentially significant cardiovascular effects, suggest α2‐adrenergic receptor agonists should only be administered to near‐term pregnant animals on a case‐by‐case basis after a risk‐benefit analysis. Muscle relaxation is reliably produced through inhibition of α2‐adrenergic receptors at spinal cord interneurons and, like benzodiazepines, α2‐adrenergic receptor agonists are frequently co‐administered with dissociative agents to counteract muscle rigidity. Involuntary muscle twitching (jactitations) has been reported in dogs, cats, and, occasionally, in horses but these effects tend to be self‐limiting. The neuroendocrine stress response is modulated by α2‐adrenergic receptor agonists. Studies involving various drugs (xylazine, medetomidine, and detomidine) consistently report decreased circulating catecholamine levels in dogs [233–236], horses [237], and cattle and sheep [238]. These reductions in epinephrine and norepinephrine levels are reversible by α2‐adrenergic receptor antagonists [236,238]. Effects on basal and induced cortisol levels in animals are more variable. Reduced cortisol concentrations have been reported after medetomidine or dexmedetomidine administration in dogs [233,235,239]. However, other studies in dogs [234,240], horses [237], and cattle and sheep [238] report no suppression of cortisol activity. In humans, a meta‐analysis of over 4800 patients found that dexmedetomidine infusion during the perioperative period inhibited release of epinephrine, norepinephrine, and cortisol and concluded that the drug attenuated perioperative stress and inflammation and protected immune function in surgical patients [241]. Glucose regulation is also impacted by α2‐adrenergic receptor agonist administration. Elevations in serum glucose are primarily a result of activation of α2A‐adrenergic receptors in pancreatic β‐cells suppressing insulin release, though stimulation of glucagon release by pancreatic α‐cells promoting gluconeogenesis has also been suggested [242–244]. Transient hyperglycemia has been reported in horses and cattle after xylazine [245,246], and in dogs after medetomidine [236] and dexmedetomidine [240]. In dogs, hyperglycemic effects induced by medetomidine were reversed with the α2‐adrenergic receptor antagonists yohimbine and atipamezole [236], and hyperglycemia was prevented when dexmedetomidine was co‐administered with the peripheral α2‐adrenergic receptor antagonist, vatinoxan (MK‐467) [240]. Clinically, α2‐adrenergic receptor agonist‐induced hyperglycemia does not typically exceed the renal threshold for glucose (~180 mg/dL) and is not associated with glucosuria [51]. A search of the veterinary literature reveals that α2‐adrenergic receptor agonists have been administered to virtually every species. For more detailed information on clinical use of these drugs in specific species, including doses, the reader is directed to species‐specific chapters in this text and elsewhere. While differences in species sensitivity tend to be more significant for drugs that are less selective/less potent (i.e., xylazine) compared to those that are more selective/more potent agents (i.e., medetomidine, dexmedetomidine), the approximate ranking of species according to α2‐adrenergic receptor agonist sensitivity from most sensitive to least sensitive is: goats > sheep, cattle > camelids > horses, dogs, cats > pigs > small laboratory animals. While α2‐adrenergic receptor agonists have not historically been classified as controlled substances, the regulatory considerations regarding these drugs, notably xylazine, are rapidly evolving. In North America, illicit fentanyl adulterated with xylazine has been identified as an emerging threat to human health, and some jurisdictions are moving to make xylazine a scheduled drug. The impact of these regulatory changes on large animal veterinarians remains to be seen. While xylazine is approved for use in dogs and cats and was historically widely used in these species, the more selective and potent α2‐adrenergic receptor agonists, medetomidine (a racemic mixture of both levomedetomidine and dexmedetomidine) and dexmedetomidine (the pharmacologically active S(+)‐enantiomer of medetomidine), have largely supplanted xylazine in small animals. Romifidine is also approved for use in dogs and cats in some countries. The α2‐adrenergic receptor agonists have wide‐ranging clinical applications in dogs and cats. Even with low doses, caution is advised in patients with cardiac disease (especially those with systolic dysfunction), bradyarrhythmias, dehydration, hypovolemia, or sepsis due to significant hemodynamic effects. Combination with other sedatives, analgesics, or anesthetics will exacerbate CNS, cardiovascular, and respiratory depressant effects, and diligent titration of both injectable and inhalant anesthetics is necessary even in healthy patients. As noted previously, stimulation may cause sudden arousal in an animal that is seemingly well sedated with an α2‐adrenergic receptor agonist. Common clinical applications are listed in Box 22.1.
22
Sedatives and Tranquilizers
Introduction
Phenothiazines
Acepromazine
Mechanism of action and indications
Pharmacokinetics
Pharmacodynamics
Cardiovascular system
Respiratory system
Central nervous system
Other effects
Clinical considerations
Dogs and cats
Horses
Pigs
Butyrophenones
Azaperone
Benzodiazepines
Diazepam, midazolam, and zolazepam
Mechanism of action and indications
Pharmacokinetics
Pharmacodynamics
Cardiovascular system
Respiratory system
Central nervous system
Clinical considerations
Dogs and cats
Horses
Other species
Benzodiazepine antagonist
Flumazenil
α2‐Adrenergic receptor agonists
Xylazine, detomidine, romifidine, medetomidine, and dexmedetomidine
Mechanism of action and indications
Drug
α2:α1 adrenergic receptor binding ratio
Imidazoline receptor activity
Agonists
Xylazine
160:1
Noa
Detomidine
260:1
Yes
Romifidine
340:1
Yes
Medetomidine
1620:1
Yesa
Dexmedetomidine
1620:1
Yesa
Antagonists
Tolazoline
4:1
Yes
Yohimbine
40:1
Noa
Atipamezole
8526:1
Yesa
Vatinoxan
105:1b
Yesc
Pharmacokinetics
Pharmacodynamics
Cardiovascular system
Respiratory system
Central nervous system
Gastrointestinal system
Genitourinary system
Other effects
Clinical considerations
Dogs and cats
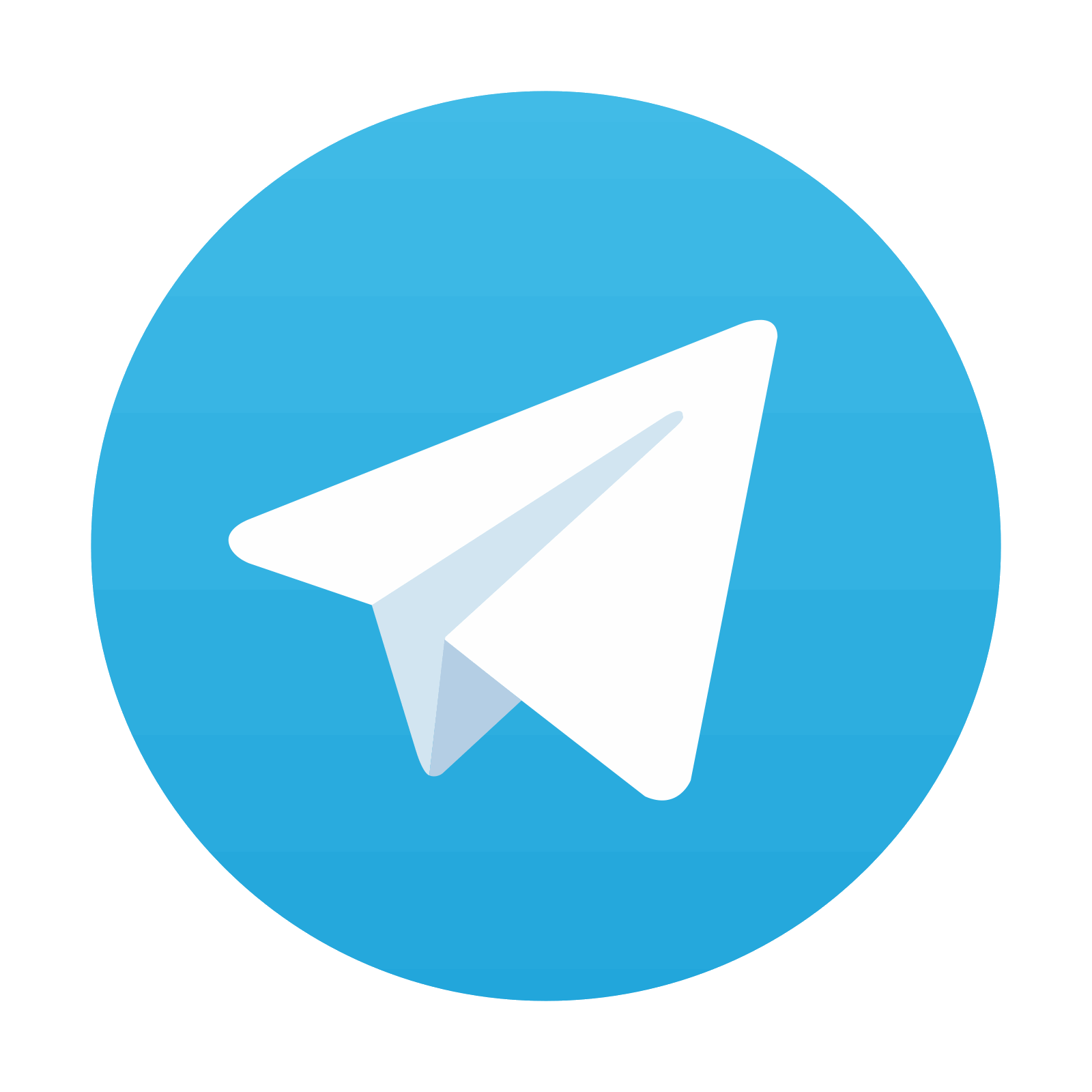
Stay updated, free articles. Join our Telegram channel
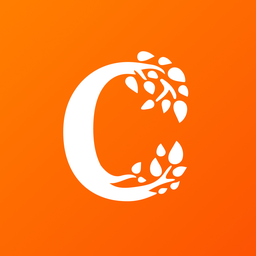
Full access? Get Clinical Tree
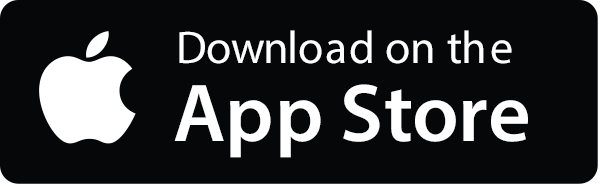
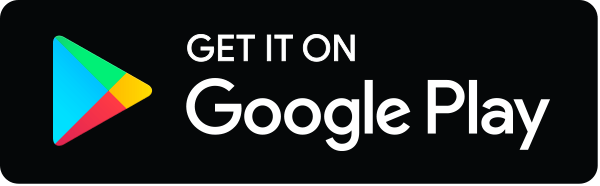