6 Rinderpest Virus 1St James’s University Hospital, Leeds, UK; 2Animal Health Rinderpest (from the German ‘cattle plague’) was a disease of significant veterinary importance affecting all species of the even-toed ungulates (Artiodactyla). The host range included cattle (both Bos taurus and Bos indicus), but also extended to small ruminants and exotic wildlife, including buffalo, giraffe, deer, kudu, wildebeest and African warthog. The geographical distribution of rinderpest reflected the history of the disease. Outbreaks attributed to the disease were first recorded in the 4th century in Asia, from which it spread to Europe by movement and transportation of cattle and eventually, in the 19th century, to Africa by colonization with concomitant introduction of domestic cattle. By the 20th century, it was endemic in Africa, Asia, the Middle East and the Indian subcontinent. Rinderpest was one of the most economically devastating veterinary diseases. The severity of a European epidemic in the 18th century led to the establishment of the first veterinary college (in Lyon in 1761) as a direct response to control rinderpest by training people as veterinarians. The Great African Pandemic in 1885 alone caused mortality rates of up to 90% in both domestic stock and wildlife, and nearly led to the extinction of the Masai people. A second epidemic from 1979 to 1983 caused the death of over 1 million cattle and it has been estimated that within this period in Nigeria alone more than 500,000 cattle died, causing an economic loss of US$1.9 billion (FAO, 2002). In response to the ongoing rinderpest outbreaks in Africa, the Pan African Rinderpest Campaign (PARC) for the control of the disease was initiated. In 1984, the United Nation’s (UN) Food and Agricultural Organization (FAO) launched the Global Rinderpest Eradication Programme (GREP) with the aim of promoting international coordination and the global eradication of rinderpest, verifying freedom from rinderpest and providing technical guidance to achieve these goals. The availability of a single dose, live attenuated vaccine (‘the Plowright vaccine’) that could induce lifelong immunity and the development of appropriate companion diagnostic assays were key factors in the eradication of rinderpest. Serological surveillance following vaccination campaigns using standardized assays (originally an indirect ELISA and subsequently a competitive monoclonal antibody (mAb)-based ELISA) allowed the testing of 1000s of samples in the regional laboratories alongside support from national laboratories across Africa, the Middle East and Asia. Furthermore, support was also available for both technology transfer and training from the World Reference Laboratories within the EU. Technological developments in diagnostic tools led to the replacement of the indirect ELISA for sero-monitoring with an advanced mAb-based competitive ELISA with greatly increased sensitivity, specificity, reproducibility and standardization. Epidemiological studies were supported by the development of technologies such as genetic typing of viruses by reverse transcription PCR, allowing the molecular characterization of virus isolates, and further lineage differentiation enabled assessment of geographical origins of new outbreaks (Forsyth and Barrett, 1995). A rapid diagnostic chromatographic strip test for the pen-side diagnosis of rinderpest, crucial in the final stages of GREP, was also developed. Based on this and through concerted efforts by the participating members of GREP, the programme finally came to fruition in 2011 when rinderpest was declared eradicated worldwide (FAO, 2011; Roeder 2011a, 2011b). Rinderpest was caused by the rinderpest virus (RPV), a member of the Morbillivirus genus. Peste des petits ruminants virus (PPRV), canine distemper virus (CDV), measles virus and phocine distemper virus (PDV) are other members of this genus. Morbillivirus virions are generally spherical and approximately 150–300 nm in diameter. They have a lipid-containing envelope that is derived from the host cell membrane on budding. These lipid envelopes contain spike-like glycoprotein protrusions of approximately 8–12 nm in length, spaced 7–10 nm apart, ‘studded’ across their surfaces called peplomers. Inside the lipid envelope the virions contain a coiled helical nucleocapsid that is 13–18 nm in diameter and up to 1000 nm in length with a pitch of 5.5–7 nm, depending on the genus (Fig. 6.1). The morbilliviruses are characterized by a single-strand negative-sense RNA genome that is 15,882 nucleotides in length. Each virion contains six structural proteins: the three nucleocapsid-associated proteins (N, an RNA-binding protein; P, a phosphoprotein; L, a large protein with characteristics of a polymerase protein); M, an unglycosylated matrix protein located under the virus envelope; and two glycosylated envelope proteins, namely the fusion (F) and attachment (H) proteins. The two virus-encoded non-structural proteins, C and V, are thought to play roles in the virus replication cycle. These two non-structural proteins may be essential for pathogenesis in vivo (Kato et al., 1997; Escoffier et al., 1999), although they can be dispensed with in vitro (Baron and Barrett, 2000). Fig. 6.1. Schematic of the morbillivirus virion. Of all the morbillivirus proteins, the P protein is antigenically the least conserved (Sheshberadaran et al., 1986) and at the nucleotide level is the most variable gene in the genome. The functioning of the P protein in virus transcription and replication has been inferred from its ability to form distinct complexes with the L and the N proteins during transcription and replication of the genome RNA (Horikami et al., 1992). However, the level of P gene expression seen in infected cells, far in excess of its representation in virions, indicates that as well as functioning in RNA synthesis it is also involved in nucleocapsid assembly. Importantly, the two non-structural proteins, C and V, are produced from the P open-reading frame (ORF) using alternate mechanisms: C is produced following RNA translation initiation at a second in frame AUG start codon within the P gene, while V is generated following RNA editing within the P ORF to produce a truncated protein. The production of these proteins highlights an evolutionary mechanism by which these viruses maximize coding capacity (Bellini et al., 1985). The morbillivirus C protein has been shown to co-localize with the N, P and L proteins in infected cells, which suggests a role in viral transcription (Sweetman et al., 2001). A recombinant RPV lacking the ability to produce the C protein was found to be defective for growth in a number of cell lines, although the levels of genome and antigenome RNAs produced were comparable to the parent virus. The absence of C from RPV was shown to affect mRNA transcription, suggesting a possible role in initiation, extension or termination of transcription (Baron and Barrett, 2000). A further function identified for the C protein is in counteracting the host response to virus infection through blocking of innate immune responses. A number of paramyxoviruses subvert the host cell’s immune response to infection by blocking interferon (IFN) signaling at different stages and in many viral infections this has been attributed to the C protein. The V protein is produced as a result of a pseudotemplated transcription where a G residue is added to the P gene mRNA during transcription (Lamb and Kolakofsky, 2001). In RPV infections, the V protein interacts with free N protein and is distributed throughout the cytoplasm (Baron and Barrett, 2000) and RPV lacking the V protein, in the presence or absence of the C protein, shows greater genome and antigenome production than wildtype virus (Baron and Barrett, 2000). This points to an influence of the V protein on replication rates and may therefore play a role in viral pathogenesis. The role of the accessory proteins of negative strand viruses is reviewed in Gerlier and Lyles (2011). The M proteins of all the morbilliviruses sequenced to date have a single ORF of 1005 nucleotides (Mahapatra et al., 2003). The differences seen in the lengths of the M genes of morbilliviruses arise from the presence of a long untranslated GC-rich region present at their 5′ ends. These GC-rich regions are of unknown function, but for RPV, removal of this region seems to have little effect on virus growth in vitro (T. Barrett, personal communication). Many studies have suggested a role for the M protein in virus budding. In this role the M protein is thought to bring together the RNP complexes, which accumulate in the cytoplasm of the infected cell, and the viral glycoproteins, which, through their synthesis on the rough endoplasmic reticulum (RER), localize to the plasma membrane of the cell (Stricker et al., 1994; Wild et al., 1995). This function is conserved among the negative-stand RNA viruses and within the morbillivirus genus the M gene is the most conserved gene (Limo and Yilma, 1990; Curran et al., 1992; Baron et al., 1994). The polymerase protein performs a number of different functions during the replication of RPV. It acts as an essential subunit of the viral polymerase complex, through interactions with the P and N proteins. L possesses transcriptase and replicase activities as well as acting to cap, methylate and polyadenylate viral mRNAs. Within the morbilliviruses three highly conserved domains that are separated by two hinge regions have been identified. By incorporating c-myc tags at the variable hinge regions of the MeV L protein, it has been shown that these regions vary in their tolerance to insertion and spatial displacement. Abrogation of polymerase function was seen with the insertion of a c-myc tag into the N-proximal hinge region that was attributed to the possible reorientation of a catalytic domain. The second hinge region towards the C-terminus of the L gene is more conserved among the morbilliviruses, but insertion of the tag at this position had no effect on polymerase function. Further studies using the green fluorescent protein (GFP) ORF showed that it was possible to insert the entire GFP ORF into the second hinge region without abolishing L protein function. The incorporation of the GFP-containing polymerase into a recombinant virus did not seriously debilitate its growth in vitro or in vivo. This observation may have many applications for the study of the polymerase unit and virus pathogenicity through the ability to trace the virus in tissues (Duprex et al., 2002; Brown et al., 2005). Rinderpest was transmitted to susceptible animals via droplet spread through close contact with contaminated nasal/ocular secretions as well as faecal contamination. The incubation period varied between 3 and 15 days, depending on the strain, route of entry, infective dose and herd susceptibility. This prodrome was followed by an acute, febrile period and then by mucopurulent discharge, erosions on the gum and tongue and finally shooting diarrhoea. Necrotic-haemorrhagic erosions in the intestines, enlarged lymph nodes, tachycardia and dehydration were often observed. Death occurred within 6–12 days after the onset of fever. Subacute reactions and atypical forms displayed mild clinical signs and low mortality, whereas peracute reactions characterized by a sudden onset and death (within 2–3 days) were occasionally observed in young calves. Morbidity and mortality rates varied, depending on the virulence of the strain involved, but could be up to 100% in a group of naive animals. Once recovered, the affected animals had generally developed a life-long sterilizing immunity. Small even-toed ungulates such as goats and sheep rarely presented with pyrexia, anorexia and intermittent diarrhoea, but this was species-dependent and most African goats and sheep exhibited subclinical infections. Rinderpest in European pigs was characterized by pyrexia, conjunctivitis, buccal erosions and death, but in African, Asian and Middle Eastern breeds was subclinical. Pathological features of rinderpest have been described in detail (Brown and Torres, 1994; Brown et al., 1996; Anderson et al., 1996; Taylor et al., 2005). For the purpose of this chapter, the most common features of rinderpest pathology are summarized here. Perhaps in the most classical form, in cattle and buffalo, rinderpest presented as emaciation and dehydration with signs of diarrhoea and mucopurolent discharge. Congested conjunctiva could be oedematous and corneal ulceration may have occurred. The oral cavity was often affected, but depending on the infecting strain and stage of disease this varied from pinhead or larger necrotic foci to extensive necrosis and gum erosions. The soft palate, pharynx and upper oesophagus might also be affected by necrosis. In some cases, necrosis also occurred in the pillars of the rumen, but with no effect on other areas of the rumen or reticulum. Erosions and haemorrhages might occur in the omasum. The abomasum might also be affected, with evidence of congestion, petechial haemorrhage and oedema. Peyer’s patches might show signs of white necrotic foci, but apart from necrosis and sloughing in neighbouring regions, the small intestines usually remained unaffected. The large intestines might be affected by the presence of blood and blood clots in the lumen; intestinal walls might appear congested and affected by oedema and erosions, which often occurred in the upper colon. The characteristic ‘tiger or zebra striping’ was often seen in the colon and in rectal mucosae in animals with late stage disease. Lungs might be affected by emphysema, congestion and secondary bronchopneumonia. The kidneys were usually unaffected, with some congestion notable in the medulla. However, severe desquamation of the epithelium in the urinary bladder was caused by infiltration of erythrocytes into the underlying stroma. RPV and other morbilliviruses are highly lymphotrophic and, as such, following RPV infection tissues of the lymphatic system were often severely affected; enlargement of the spleen and lymph nodes were observed with the most damage in the mesenteric lymph nodes and lymphoid tissues associated with the intestines; the gall bladder might be affected by petechiae and ecchymoses. RPV was also epitheliotrophic and so also caused extensive infection within the epithelia of the alimentary, upper respiratory and urogenital tract. Severity of infection and presence at post-mortem findings depended on the strain involved and time of death after the onset of illness. Carcasses of animals that had died early after infection and before the onset of the diarrhoeic phase often presented without the rinderpest-associated soiledness and mucupurolent discharges. Less pronounced post-mortem findings were recorded for sheep and goats, as well as pigs (Fig. 6.2A). Haematological and biochemical changes were also detected during rinderpest infections. The lymphotrophic nature of RPV caused total leucocyte counts to reduce sharply during the prodromal fever phase of infection and then recovered to normal levels if the animal managed to clear the infection and survive. This drop was attributable to the destruction of both T- and B-lymphcoytes, with a sharp decline during the prodromal fever, which slowly recovered in surviving animals. Research studies demonstrated that RPV grew well in B-cells, CD4+ and CD8+ alpha/beta T-cells, and gamma/delta T cells with no preference for a particular phenotype (Anderson et al., 1996). Monocyte numbers were not affected and neutrophil numbers only appeared affected in fatal cases. Eosinophils appeared to follow the lymphocytic pattern, whereas basophil numbers only dropped in fatal cases after onset of the prodromal fever. Increases of erythrocytes in fatal cases were linked to severe cases of dehydration (Fig. 6.2B). Various biochemical changes (such as loss of water and electrolytes, metabolic acidosis, hypoglycaemia) were also reported in rinderpest infections (Anderson et al., 1996). Fig. 6.2. Pathobiology of rinderpest. Characteristic pathological features: (A) emphysema of the lung; (B) inflamed and haemorrhagic serosal and mucosal surfaces of Peyer’s patches; (C) zebra striping of the intestines – haemorrhages along the crests of the mucosae gave rise to the characteristic striped pattern, which appeared red when recent and green when older; (D) severe congestion and haemorrhage of the fourth stomach; (E) haematological changes during infection (after Anderson et al., 1996). [(A) courtesy of Dr Ashley Banyard; (B–D) courtesy of Jenny Ryder and Professor John Anderson.] Diagnosis of rinderpest took place firstly in the field or at pen-side by thorough examination of suspect animals and carcasses within a herd and the presence of the tell-tale three ‘Ds’, discharge, diarrhoea and death (Fig. 6.3A). However, many of the clinical signs observed in rinderpest could also be attributable to other viral diseases of livestock, including peste des petits ruminants (PPR), foot and mouth disease (FMD), bovine viral diarrhoea (BVD), Bluetongue (BT) and malignant catarrhal fever (MCF), whose geographical distribution often overlapped with that of RPV. In addition, during the last rinderpest outbreaks in the 21st century, the presence of mild forms of rinderpest made field diagnosis more problematic. For rinderpest to be diagnosed conclusively, it needed to be confirmed in a laboratory. This could be achieved directly by the detection of antigen in the samples from suspect animals, including secretions/excretions and tissues, by the isolation and identification of the strain in question and/or by the detection of specific antibodies in the sera from suspect animals (Fig. 6.3B). For a successful detection of rinderpest antigen in secretions/excretion or tissues, the selection of the right sample and its transportation was crucial. Scientific research had revealed that a high viral load was found in ocular and nasal secretions, gum debris, blood and tissues (including spleen, lymph node and tonsil) while the animal was in the pyrexic stage of the disease. To avoid the rapid dissociation of the virus due to exposure to light, heat and extreme pH, all samples had to be transported on wet ice in a secure vessel such as an icebox. The timing of sampling was crucial. Highest viral loads were found to be present during the prodromal fever and the erosive mucosa phase, with viral shedding decreasing with progression of illness. For sampling to be most effective, it therefore had to take place during the prodromal fever and early onset of the erosive-mucosa phase. Direct diagnosis of RPV was achieved in the laboratory by various methods, which can be grouped into classical and new as defined by the methodologies adopted prior to and after the initiation of rinderpest eradication programmes. Classical rinderpest antigen detection included the following methods. This method was believed to be the gold standard for the definitive diagnosis of the presence of RPV, especially for regions with previously unknown rinderpest infections. For virus isolation, several prerequisites were vital, including the availability of laboratory facilities with class II laminar flow cabinets, incubators, auto-claves, centrifuges, filtration equipment, microscopes and roller apparatus. Virus isolation was achieved by growing the suspect virus in susceptible cell culture, traditionally – in the case of RPV – primary calf kidney cells. Calf kidney cells had to be established from a donor calf, dissected and processed under sterile conditions to obtain primary cell cultures of actively dividing cells. The cells were eventually grown in roller bottles and placed on roller drums, allowing cells to continue growing without contact inhibition. Primary cell cultures were then used to produce secondary bovine kidney cells for virus isolation. For virus isolation, field specimens including blood and solid tissues were prepared to yield a buffy coat suspension from blood samples and cell suspensions were prepared from ground tissues ready for inoculation into the roller tubes. After inoculation of the samples, the medium was removed from the cell layers and replaced with maintenance medium supplemented with or without rinderpest immune serum. This procedure was carried out over a period of 2 weeks and if no virus was detected by the appearance of rinderpest-specific cytopathic effects (CPE), the cultures were blind passaged and re-examined. Rinderpest CPEs could be identified by the typical appearance of densely granular syncytia in the cultures with no added rinderpest immune serum and by the absence of CPE in the cultures with added immune serum. This type of isolation not only allowed the detection of rinderpest in a sample, but also the generation and harvesting of viral material for further analysis. Drawbacks of this technique included the requirement of tissue culture facilities and highly skilled staff, the time-consuming nature of the test and the fact that PPR caused similar infection in bovine kidney cells, thus preventing a differential diagnosis.
and Veterinary Laboratories Agency, Weybridge, UK
6.1 Introduction
6.2 Rinderpest Causative Agent
6.3 Clinical Signs
6.4 Pathology
6.5 Diagnosis
6.5.1 Sample collection for rinderpest antigen detection
6.5.2 Antigen detection
Virus isolation
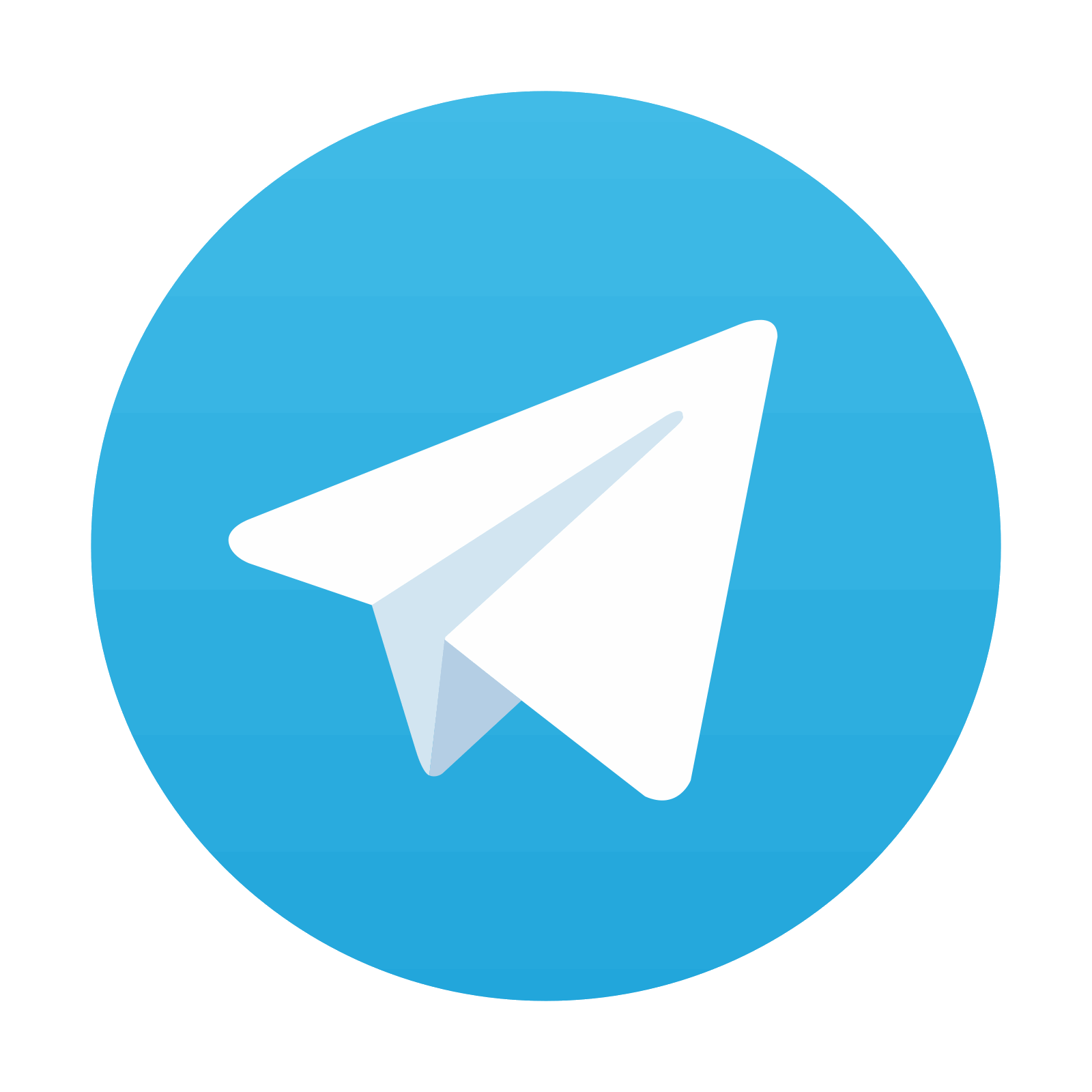
Stay updated, free articles. Join our Telegram channel
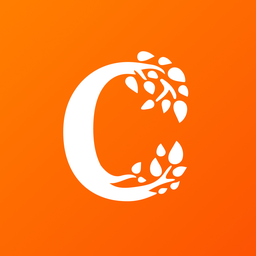
Full access? Get Clinical Tree
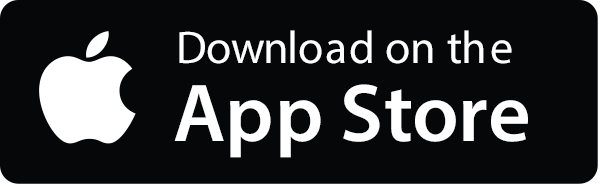
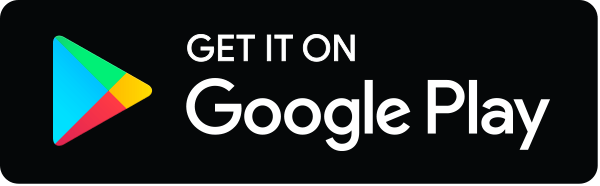