Fig. 9.1
Molecular clock of the suprachiasmatic nucleus (SCN). A simplified view of the molecular circadian clock. The mammalian clock is composed of a negative feedback loop involving clock genes clock, bmal1, per1, per2, cry1, and cry2. Transcription factors CLOCK and BMAL1 interact and activate per and cry gene expression by binding to an E-box in their promoter regions. PER and CRY proteins heterodimerize, translocate to the nucleus, and inhibit Clock/Bmali-induced gene expression. After the PER/CRY complex is degraded, the cycle starts again. The alternation between the activation and the repression has a cycle length of about 24 h. (From Yu and Weaver 2011)
In addition to this core loop, there is a secondary negative feedback loop, in which REV-ERBα and ROR (both nuclear receptors) act to stabilize Bmal1 transcription, thereby contributing to clock precision and robustness (Reppert and Weaver 2001, 2002; Ueda et al. 2005; Ukai and Ueda 2010). These transcription–translation feedback loops, present within individual cells, provide the basic mechanism of circadian oscillation. Entrainment to local environmental time is thought to result from adjustments of various steps in these oscillating feedback loops.
An important property of the SCN as a network is the synchronization of the individual oscillator cells. Loss or mutation of specific clock genes alters period length (Cry1, Cry2, Per1, Per3) and decreases (Clock, Per2) or causes immediate loss (Bmal1) of circadian rhythmicity under constant conditions. Cry1/Cry2 and Per1/Per2 double mutant animals lose circadian rhythmicity immediately under constant conditions, confirming the importance of these genes in the clock mechanism (Vitaterna et al. 1999; Bae et al. 2001; Zheng et al. 2001). CRY1 and CRY2 appear to be necessary for the development of intercellular networks that subserve coherent rhythm expression in adult SCN. There are developmental changes in SCN circadian rhythms in Cry1 and Cry2 double deficient (Cry-null mice). Rhythms in electrical activity and PER expression were detected in both neonatal and adult SCN individual cells; however, at the level of the SCN as a whole, circadian rhythms are found in only neonatal not adult SCN. Importantly synchronized circadian rhythms in adult Cry-null SCN were restored by coculture with neonatal, but not juvenile, SCN (Ono et al. 2013).
Present Goal
The molecular revolution enabled substantial exploration of the mechanisms underlying SCN clock function. Our goal is to review current understanding of how the individual “clock” cells of this small nucleus in the hypothalamus encode circadian time and how they work together to set the schedule of daily behavioral and physiological responses throughout the entire body. We discuss the importance of the interconnections among the varied cells and clusters of cells of the SCN, to explore how SCN circuitry underlies its master clock function. We also point to some of the consequences of failures in brain clock function. More specifically, we consider circadian oscillation when molecular elements of the feedback cycle are missing, when key subregions of the SCN are separated from each other, and the consequences of circadian disruptions associated with jet lag.
9.2 SCN Intrinsic Anatomy
The SCN is a bilaterally symmetrical structure located at the base of the brain, in the anterio-ventral hypothalamus situated dorsal to, and extending into, the optic chiasm (Fig. 9.2). The SCN arises from the ventral diencephalic germinal epithelium as a component of the periventricular cell groups (Altman and Bayer 1986). It has a rostrocaudal extent of approximately 600 μm in the mouse and the rat (van den Pol 1980; Abrahamson and Moore 2001), 650 μm in the hamster (Card and Moore 1984) and 4.7 mm in humans (Moore 1996b). It is comprised of ~10,000 neurons on each side in rodents, and ~45,000 in humans (Abrahamson and Moore 2001). The neurons in the SCN are small, about 8–10 μm of diameter, densely packed, and able to generate self-sustained oscillations of electrical activity (Welsh et al. 1995). In addition to neurons, the SCN contains glial cells of many shapes and sizes. They provide structural support to surrounding neurons, regulate ionic and neurotransmitter levels in extracellular fluid, and secrete neurotrophic factors (rev in Middeldorp and Hol 2011; Paratcha and Ledda 2008; Verkhratsky and Steinhäuser 2000 for further detail). Glial cells appear to contribute to the pacemaker function of the SCN, as mice that have a mutation in glial fibrilliary acidic protein (GFAP) have altered circadian activity rhythms in a constant light environment (Moriya et al. 2000). Most work on SCN organization has been done on material examined in the coronal plane and focused on anatomy and function in the area of its greatest extent, specifically, a caudal aspect that includes the core and shell regions. A sagittal view (Fig. 9.2) of the SCN provides a window into its overall extent.
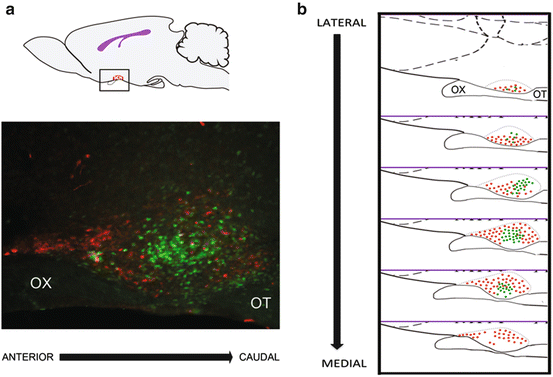
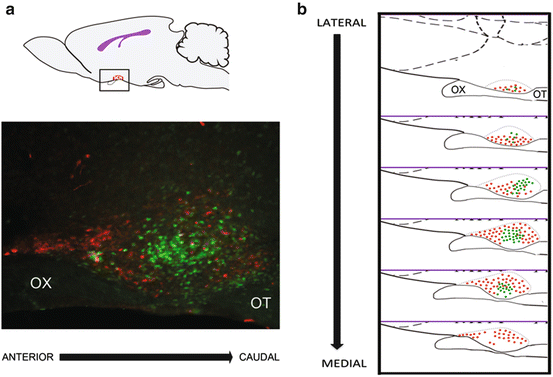
Fig. 9.2
The suprachiasmatic nucleus (SCN). (a) Sagittal view of a rodent brain (upper panel) illustrates the approximate location of the SCN in the anterior hypothalamus (red oval). The lower panel shows a photomicrograph through the mid-SCN region, in the same orientation as the cartoon with GFP:GRP cells in green and AVP in red. (b) Schematic of the SCN, in a sagittal plane, from the lateral to the medial aspect. The core region is delimited by the red dots, and the shell region is indicated by green dots. OX, optic chiasm, OT, optic tract
9.2.1 Afferents
The core SCN receives photic input from the retina through two different pathways. Photic input reaches the nucleus via the monosynaptic RHT (Moore and Lenn 1972), and indirectly through the geniculo-hypothalamic tract (GHT), which originates in NPY-containing cells of the thalamic intergeniculate leaflet (IGL) and anterior portions of the ventral lateral geniculate nucleus (vLGN) (Morin et al. 1992; Harrington 1996). In addition, the core SCN receives nonphotic input, which arrives from the serotonergic neurons of the dorsal and median raphe nuclei pathway (Moga and Moore 1997; Hay-Schmidt et al. 2003) (Fig. 9.3). The IGL and the dorsal raphe communicate information about locomotor activity (Janik and Mrosovsky 1994; Kuroda et al. 1997), and IGL may also provide metabolic information (Saderi et al. 2013).
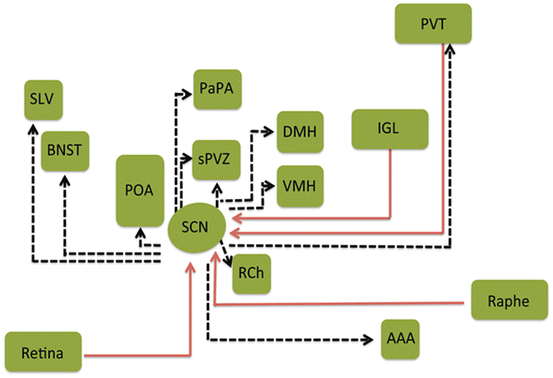
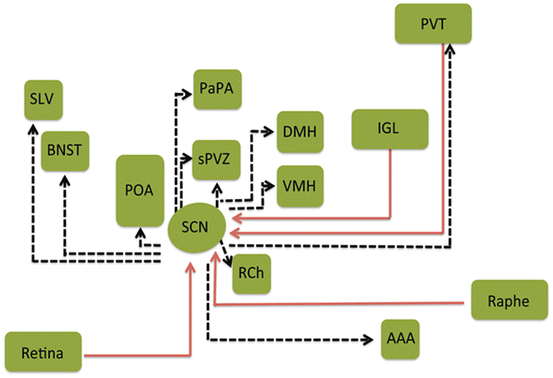
Fig. 9.3
Organization of neural inputs to and outputs from the suprachiasmatic nucleus (SCN). In the schematic illustration the SCN receives direct photic input through the retinohypothalamic tract, indirect photic input from the intergeniculate leaflet (IGL) of the thalamus, and serotonergic input from the raphe. Afferents from other hypothalamic nuclei and parts of the limbic system, among other afferents, including the hippocampus and brainstem. The SCN efferents reach the dorsomedial nucleus of the hypothalamus (DMH) and medial subparaventricular zone (sPVZ) and the lateral sPVZ. LS lateral septum, ILC infralimbic cortex, VS ventral subiculum, VMH ventromedial nucleus of the hypothalamus, ARC arcuate nucleus, LHA lateral hypothalamic area. (Modified from Morin 2013)
The shell region receives dense input from hypothalamus and limbic areas such as the ventral subiculum and limbic cortex (Leak et al. 1999; Moga and Moore 1997; Krout et al. 2002 for further details). Finally, afferents from the paraventricular nucleus of the midline thalamus are distributed throughout the SCN (Moga et al. 1995; Moga and Moore 1997). This specialization in input pathways to regionally distinct populations of cells points to functional heterogeneity within the nucleus.
9.2.2 Endocrine Signals to SCN
While the majority of studies focus on the neural afferent input to the SCN, endogenous humoral signals also act on the SCN, enabling integration in internal and external temporal information. Gonadectomy results in marked alteration of circadian behaviors in mice, including lengthened free-running period, decreased precision of daily onset of running, and elimination of early-evening but not late-night activity bouts (Karatsoreos et al. 2007; Daan et al. 1975). Androgen replacement restores these responses. The evidence suggests that the brain clock is an important site of these androgen actions. As already noted, the rodent SCN is composed of a core and a shell region. Androgen receptors (AR) are localized to the core SCN (Karatsoreos et al. 2007). Analysis of a transgenic mouse bearing two reporter molecules driven by the AR targeted to both membrane and nucleus indicates that projections of AR-containing cells form a dense plexus in the core, with their fibers exiting the SCN dorsally. Dual label immunochemistry indicates that gastrin-releasing peptide cells contain AR and that these cells express FOS after a light pulse. As is well known, increasing intensities of constant light parametrically increase circadian period, and this effect was potentiated at all intensities by gonadectomy (Butler et al. 2012). Gonadectomy reduces the FOS response after a phase-shifting light pulse, whereas androgen replacement restores the response to that seen in intact animals. Gonadectomy also increased glial fibrilliary acidic protein expression and decreased expression of the synaptic proteins synaptophysin and postsynaptic density 95 (Karatsoreos et al. 2011). Treatment of gonadectomized animals with the nonaromatizable androgen dihydrotestosterone restored glial fibrilliary acidic protein, postsynaptic density 95, and synaptophysin in the SCN and reinstated the normal pattern of responses to light. Together, the results reveal a role for androgens in regulating circuitry in the mouse SCN, with functional consequences for photic phase resetting stimuli.
AR expression is sexually dimorphic in humans and rodents, with greater expression in males than in females (reviewed in Karatsoreos and Silver 2007). Both Western blots and immunochemistry confirm that ARs are more highly expressed in male than in female mice. Gonadectomy eliminates and androgen treatment restores these sex differences, indicating that the response is dependent on circulating hormones rather than on genetic factors (Iwahana et al. 2008). Interestingly, while there is little ER alpha in the SCN, ERbeta-IR neurons are localized to the shell subdivision of the nucleus, with significantly greater numbers in females than in males (Vida et al. 2008). Treatment of either gonadectomized mice of either sex with estradiol benzoate reduced the number of ERbeta-IR neurons, with no effect of ERalpha-IR. The sexually differentiated expression and distribution of ARs and ERs in various cell populations of the SCN suggest multiple modes of hormone action within the SCN.
9.2.3 SCN Efferent Connections
Monosynaptic SCN efferents primarily reach nearby hypothalamic targets (Moore 1996a; Abrahamson and Moore 2001). The densest projections to the subparaventricular zone (sPVZ), the preoptic area (POA), bed nucleus of the stria terminalis (BNST), the lateral septum (LS), retrochiasmatic area (RCA), the arcuate nucleus (ARC), and the dorsomedial hypothalamus (DMH) come from the shell, whereas the lateral sPVZ receives most of its input from the core (Watts et al. 1987; Leak and Moore 2001) (Fig. 9.3). Limited projections to the forebrain, midline thalamus, IGL, and periaqueductal gray are also present. These direct SCN recipient areas in turn signal many other brain regions with the result that most of the brain gets rhythmic input and has daily rhythms of activity (Harbour et al. 2013; Morin 2013). Circadian rhythms are also expressed in virtually all peripheral bodily tissues. Time signals from the SCN travel to peripheral organs by the sympathetic and parasympathetic nervous systems and by circadian fluctuations in adrenal glucocorticoids (Ota et al. 2012).
9.2.4 SCN Diffusible Signals
In addition to these neural efferents, the SCN also produces diffusible signals, which represent another SCN output. When SCN donor tissue graft is encapsulated within a semipermeable membrane, circadian locomotor activity is restored (Silver et al. 1996; LeSauter and Silver 1998). The polymer capsule allows chemicals to diffuse through but does not permit fibers to exit the graft. Several diffusible signals have been studied, including prokineticin 2 (PK2) (Cheng et al. 2002; Prosser et al. 2007), transforming growth factor-alpha (TGF-α) (Kramer et al. 2001), and cardiotrophin-like cytokine (Kraves and Weitz 2006). Both PK2 and TGF-α are secreted in a circadian fashion. When infused into the third ventricle of the rat or hamster brain, they suppress locomotor activity. This may be a mechanism for supporting rest–activity cycles. While it has been suggested that diffusible signals produced by the SCN may travel to distal tissues located widely in the brain and even in the gut (Schibler and Sassone-Corsi 2002), the distance over which such signals can travel is actually not known.
Diffusible signals from the SCN serve to communicate not only with nearby extra-SCN regions, but also within the nucleus itself. Diffusible interneuronal signals have been examined in an SCN slice coculture using wild-type “donor graft” SCN to drive pacemaking (monitored by PER2::LUCIFERASE bioluminescence) in “host target” SCN deficient either in elements of neuropeptidergic signaling or in elements of the core feedback loop (Maywood et al. 2011). A hierarchy of neuropeptidergic signals underpins paracrine regulation by vasoactive intestinal polypeptide (VIP) augmented by contributions from arginine vasopressin (AVP) and gastrin-releasing peptide (GRP). Also paracrine signaling maintains circadian pacemaking in arrhythmic Cry-null SCN, deficient in essential elements of the transcriptional negative feedback loops (Ono et al. 2013). These studies indicate that paracrine neuropeptidergic signals determine cell- and circuit-level circadian pacemaking within the SCN. In summary, the pacemaking function in the in vitro coculture preparation, and in whole animal transplant experiments, is specified by the genotype of the graft, confirming graft-derived factors as determinants of the host rhythm. The next question is how the pacemaking function of the brain’s master clock’s many individual neurons is achieved, given the heterogeneity of its inputs and outputs. This can be addressed by examining the functional and spatial organization of the SCN.
9.3 Functional Organization of SCN Neurons
9.3.1 Properties and Functions of Core and Shell SCN
Early studies suggested that rhythmicity was lost if the entire nucleus was lesioned, but was sustained if any part of the nucleus survived ablation. With the advent of markers enabling identification of specific subregions of the nucleus, it became clear that the core and shell have markedly different properties. Period genes Per1, Per2, and Per3 mRNA expression are differentially regulated in the two compartments. The shell exhibits endogenous rhythmicity in Per1, Per2, and Per3 mRNA. The core compartment does not have high-amplitude rhythmic mRNA expression but has temporally gated light-induced Per1 and Per2 and high levels of endogenous non-rhythmic Per3 mRNA expression (see below for discussion of gating). These results reveal differential regulation of clock genes in the two distinct SCN regions (Hamada et al. 2001). Furthermore, lesions of the SCN core eliminate all circadian rhythms, including locomotor activity, drinking, gnawing, body temperature, and hormone secretion. Loss of these rhythms occurs even when substantial numbers of cells in the SCN shell survive the lesion (LeSauter and Silver 1999; Kriegsfeld et al. 2004). This finding is further confirmed in work on SCN slices in vitro where the ventral SCN (containing the core) is separated from dorsal SCN. In slice studies, when core and shell of mouse SCN were separated, the shell was desynchronized while the core was not, pointing to the importance of connections from the core in synchronizing neurons in the shell (Yamaguchi et al. 2003). Such findings lead to the hypothesis that cells of the core, which themselves do not appear to have high-amplitude oscillation of clock genes, are necessary to coordinate oscillation of network of cells in the shell.
9.3.2 Function of the Shell SCN
Disrupting the intercellular coupling among neurons in the SCN indicated the existence of regional differences in the periods of the oscillating small-latticed regions of the SCN in an imaging study using a transgenic rat carrying a luciferase reporter gene for Per2 (Per2::dluc) (Koinuma et al. 2013). Here, the results show that the SCN can be divided into two regions—one with periods shorter than 24 h and another with periods longer than 24 h. It appears that the topographically specific and multiphase oscillations of clock gene expression are inherent properties of the SCN. The foregoing studies point to properties of SCN networks. The robust oscillation of the SCN rests on a specific linkage of oscillations that bear a characteristic pattern of changes in spatial extent of the nucleus and changes within subregions that occur in specific temporal niches for each region: time spatial and temporal organization of the SCN. Why such heterogeneity is necessary for SCN function is discussed further below.
Such findings draw our attention to the properties of individual cells versus that of SCN networks.
9.3.3 Relation of Cells to Networks
When individual cells are dispersed, the period of the individual oscillators is much broader than that seen in cells within the intact network (Welsh et al. 1995). Within cultured explants, individual neurons express rhythms with a period of about 24 h, while dissociated neurons that are plated at low density oscillate with a much broader range of periods (Welsh et al. 1995; Liu et al. 1997; Herzog et al. 1998; Honma et al. 1998; Nakamura et al. 2001). This indicates that the period of the SCN tissue as a whole requires an interconnected networks to generate its typical coherent oscillation. Also, while dispersed SCN cells sustain rhythms, they are unstable oscillators when dispersed, indicating that they require network interactions for robust cycling (Aton et al. 2005).
9.3.4 Gating Mechanisms
As we have seen, a fundamental question with regard to the organization of circadian timing is how the SCN achieves a coherent oscillation while their constituent independent cellular oscillators express a wide range of periods. The SCN tissue shows specific modes of coupling mediated by specialized mechanisms, not seen in other tissues bearing oscillators. Furthermore, the amplitude of the SCN intracellular clock is highly responsive to and dependent on intercellular signaling. The most prominent network property of the SCN is the coupling of its cellular oscillators which then produce a coherent circadian oscillation at the level of the tissue. One might imagine that the individual SCN cells might be coupled and that the consensus output of individual oscillators is a summation of weak coupling among cells. Instead, it is clear that in each circadian cycle, activation of PER expression begins in dorsomedial SCN, and proceeds ventrally and laterally, followed by marked activation of an inner area or “cap” just dorsal to the core (Foley et al. 2011; Nakamura et al. 2001; Yan and Okamura 2002). This evidence argues strongly that SCN coupling is mediated by specific neural circuits.
One model, attractive in its simplicity, is neutral with regard to inter-neuronal coupling mechanisms and rests on the differences between core and shell properties. Specifically, the differences in oscillation between core and shell cells suggest a model that incorporates non-rhythmic “gate” cells and rhythmic oscillator cells with a wide range of periods. In this model, the gate provides daily input to oscillator cells and is in turn regulated (directly or indirectly) by the oscillator cells. Individual oscillators with initial random phases use the phase-setting information from the gate cells to self-assemble so as to maintain cohesive rhythmic output. The model explains how individual SCN cells oscillate independently and yet work together to produce a coherent rhythm (Antle et al. 2003). An extension of this model considers how the system responds when the gate cells are activated by an external stimulus, such as an entraining or phase-setting signal. Here, the model shows that exogenous triggering of the gate over a number of days can organize a completely arrhythmic system, simulating the light cue-dependent reappearance of rhythmicity in a population of disorganized, independent oscillators. A significant merit of this model is that a single mechanism (i.e., the output of gate cells) can account for not only free-running and entrained rhythmicity but also limits of entrainment, a PRC with both delay and advance zones, and the light-dependent reappearance of rhythmicity in an arrhythmic animal (Antle et al. 2007). (To intuit this scheme, imagine a row of children on swings. Even though they may start out swinging along separately, if their parents coordinate the time at which they push their offspring, they will start to swing in phase with each other). In summary, individual cells are oscillators, but the oscillation of the SCN requires network properties. While the foregoing model focuses on the relation of core to shell cells, it is also interesting to consider the relation of shell cells to each other.
Clearly the contributions of the chemical and electrical interconnections among neurons are essential to circuit-level organization; how the ensemble of oscillators work together is not known. By applying quantitative methods it is possible to deconstruct the interactions between the spatial and the temporal organization of circadian oscillations in organotypic slices from mice with circadian abnormalities. Clues to SCN network organization come from studies of mice lacking Cryptochrome genes (Cry1, Cry2), which are essential for cell-autonomous oscillation, and in other SCN of mice lacking the VPAC2 receptor, which is necessary for circuit-level integration (Pauls et al. 2014). The SCN of wild-type mice show a strong link between the temporal rhythm of the bioluminescence profiles of PER2::LUC and spatial coherence in portions of tissue. Cry-null SCN have stable spatial organization but lack temporal organization, while some VPAC2-null specimens can exhibit temporal organization in the absence of spatial organization. The results indicate that spatial and temporal organization are separable, that they may have different mechanistic origins (cell-autonomous vs. interneuronal signaling), and that both are necessary to maintain robust and organized circadian rhythms throughout the SCN. This work provides evidence that the coherent properties of the neuronal circuitry, revealed in the spatially organized clusters, are essential to the pacemaking function of the SCN.
9.4 Peptides of the SCN
While the foregoing consideration of networks indicates that diffusible signals cannot account for the phase dispersion of SCN oscillators, it is nevertheless the case that SCN cells can release peptidergic signals. The consideration of SCN peptides points to another level of organization of neurons. It is well established that SCN neurons are comprised of many different peptidergic cell types. These are not dispersed through the nucleus, but instead are organized within clusters of similar cells (Fig. 9.4). However, there is evidence that regionally clustered peptidergic cells have different free-running periods. For example, the period of AVP-releasing rhythm in the dorsal SCN is shorter than that in the ventral SCN (Noguchi et al. 2004; Noguchi and Watanabe 2008). Such findings point to the potential role of paracrine/autocrine signals.
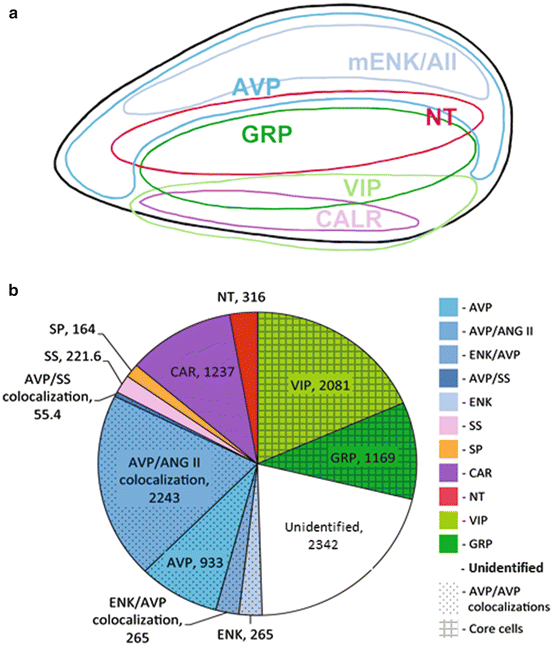
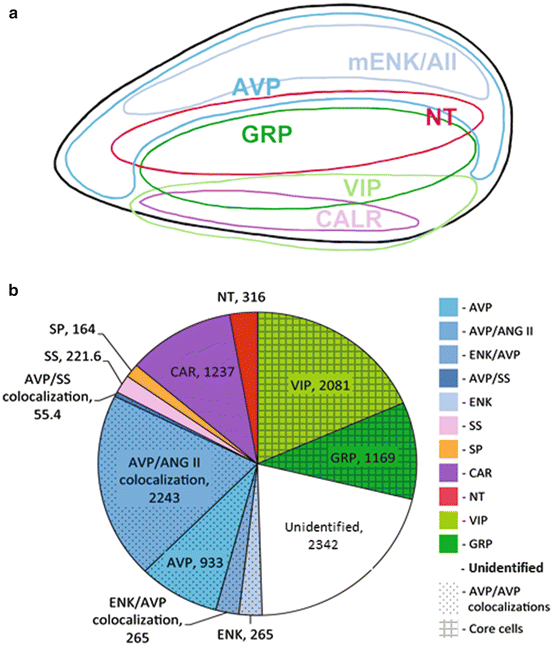
Fig. 9.4
Chemoarchitecture of the suprachiasmatic nucleus. (a) The approximate location of clusters of cells bearing peptides of the core and shell regions is seen in sagittal section view. Cells in the core contain VIP, GRP, and CALR and small populations of NT and SP (not shown). Cells in the shell bear AVP and ENK among other peptides. (Modified from Yan et al. 2007). (b) The approximate numbers of peptidergic cells of each type are depicted in the “color wheel” based on single- and double-label studies. Note that many peptides are co-expressed in a given cell type and that approximately 20 % of cells remain to be identified (modified from Moore et al. 2002)
Typically, the core and shell are characterized by different peptidergic cell types. In most species, AVP cells are located in the shell and VIP cells in the core. That said, cells of the core, and those of regions intercalated between distinctly core and shell areas are somewhat more variable among species—likely associated with adaptive species differences. A sense of the relative numbers of SCN cells bearing reasonably well-studied peptidergic phenotypes is provided in Fig. 9.4b and highlights that fact that a substantial fraction of cells remain unidentified (based on data in Moore et al. 2002). In fact, somewhat surprisingly, a study using ultrahigh performance tandem mass spectrometry produced a list of 102 endogenous peptides in the rat SCN, including 33 previously unidentified peptides, and 12 peptides that were post-translationally modified (Lee et al. 2010). To date, research has focused on a few SCN peptides. We present an overview of this work as the peptidergic phenotypes of the SCN and their specialized properties have been amply reviewed (Antle and Silver 2005; Mohawk and Takahashi 2011; Slat et al. 2013).
9.4.1 AVP
AVP-containing cells, lying in the shell region of the SCN, represent about a third of the cell population in the SCN in the rat (Abrahamson and Moore 2001; Moore et al. 2002). AVP release from SCN neurons is under circadian control and Clock mutant mice lack these AVP rhythms (Jin et al. 1999; Silver et al. 1999). In the cerebrospinal fluid, there are SCN-dependent circadian fluctuations of AVP levels with a morning peak and evening trough (Noguchi et al. 2004). AVP serves in the amplification and synchronization of the endogenous rhythmicity of the clock itself. Electrical activity of the SCN is highest during the day, when AVP release is high. Consistent with these findings, SCN neurons generally increase their firing rate following the application of AVP (Ingram et al. 1996). This clock-controlled neuropeptide is thought to play an important role in transmitting the output of the circadian clock to targets outside the SCN (Buijs and Kalsbeek 2001). For example, in the common vole, vasopressin in the SCN is correlated with the expression of circadian locomotor behavior (Jansen et al. 2007). Animals deficient for AVP or its receptor (V1a receptor) have rhythms with attenuated amplitudes (Li et al. 2009). AVP-deficient Brattleboro rats display low-amplitude daily rhythms in sleep–wake, body temperature, plasma melatonin, and SCN firing rates (Ingram et al. 1996). Mice lacking the V1aR show diminished rhythms (Robinson et al. 1988).
Coculture experiments in which diffusible signals are effective but synaptic connections are absent suggested another role for AVP in the SCN. Blocking AVP receptors does not abolish SCN rhythms in vitro (just as Brattleboro rats continue to display circadian rhythms). But in the absence of AVP receptors, wild-type SCN tissue in cocultures fails to restore rhythms to VIP-deficient SCN (Maywood et al. 2011). It is possible that AVP normally amplifies SCN rhythms and, when VIP signaling has been compromised, AVP can act as a weak synchronizing agent to coordinate the rhythms among the circadian cells of the SCN, perhaps acting through other receptors. It had been shown in early studies of the rat SCN that the oscillators in ventral and dorsal AVP cells contribute differently to period length; dorsal cells have shorter period length while those with longer periods are in the ventral aspect (Noguchi et al. 2004). Because the period of activity in the ventral SCN is approximately that of the SCN as a whole, it has been suggested that the oscillation of the shell cells is entrained by cells in the core area.
9.4.2 VIP
VIP is one of the best understood SCN peptides—because studies of mutant mice in which VIP is absent fit well with work on mice lacking the VIP receptor (VPAC2R) (Piggins and Cutler 2003; Vosko et al. 2007). VIP cells constitute about 24 % of the cells in the nucleus (Abrahamson and Moore 2001; Moore et al. 2002; Atkins et al. 2010), and is the most prevalent neuropeptide within the core area: its receptor is widely distributed in the SCN (Usdin et al. 1994; Kalamatianos et al. 2004; Kallo et al. 2004). VIP acts at VPAC2Rs to depolarize SCN neurons by closing potassium channels. VIP is released with circadian rhythmicity under LD conditions, but that rhythm is abolished in constant darkness suggesting a role for VIP in the maintenance of circadian rhythms with respect to environmental lighting (Francl et al. 2010; Shinohara et al. 1998). VIP is inhibited by serotonergic activation, suggesting a possible mode for the attenuating action of 5-hydroxytryptamine (5-HT) receptors in SCN photic signaling (Francl et al. 2010). In addition, VIP can phase-shift circadian rhythms of locomotor behavior and of firing rate of SCN neurons as well as clock gene expression, similar to the action of environmental light. Mutant mice lacking VIP or the VPAC2 receptor have weak behavioral rhythms with multiple period components. In SCN slices from these mutant mice, neuronal firing and clock gene rhythms are suppressed, largely due to desynchronization among cells (Maywood et al. 2011). However, daily application of a VIP agonist to mutant SCN cultures restores synchrony (Aton et al. 2005).
In the intact animal, there is a dose-dependent effect of VIP that is specific to time of day. VIP maximally delays circadian rhythms in the SCN around subjective dusk (Reed et al. 2001; An et al. 2011). Around the transition to dawn, VIP produces small-phase advances. When applied daily, VIP entrains the SCN in a slice preparation (An et al. 2011). Using a coculture preparation Maywood et al. (2011) showed that a wild-type SCN slice could restore circadian oscillation in a VIP-deficient SCN slice. Thus it appears that VIP can diffuse out of the SCN and is both necessary and sufficient for sustained SCN rhythms. However, wild-type SCN also restores circadian rhythms to VPAC2R-deficient SCN, though slowly. However, VIP is not the only factor capable of producing such synchronization by paracrine signals.
9.4.3 GRP
GRP is produced by approximately 10 % of SCN neurons of the core region (Abrahamson and Moore 2001; Antle et al. 2005; Atkins et al. 2010; Moore et al. 2002). The GRP receptor (BBR2) mRNA appears throughout the SCN, with more in the dorsal SCN (Aida et al. 2002; Karatsoreos et al. 2006). In some ways, GRP function in the SCN overlaps with VIP. In fact, about some VIP cells in SCN also express GRP mRNA in the rat (Kawamoto et al. 2003).
GRP has been implicated in the transmission of photic information to the SCN. Anatomical evidence indicates that the cell somata of GRP neurons of the core SCN extend fibers into the shell (Silver et al. 1999; Karatsoreos et al. 2004; Drouyer et al. 2010), where they communicate with other types of SCN neurons. GRP application induces coordinated circadian rhythms in SCN slices from mice deficient in VPAC2R (Brown et al. 2005; Maywood et al. 2006). GRP, acting through BB2 receptors, induces Per1, Per2, and c-fos expression in the shell SCN (Aida et al. 2002). Like VIP neurons, GRP neurons receive retinal input and respond to nocturnal light with increased transcription of c-fos and the Period genes (Bryant et al. 2000; Karatsoreos et al. 2004). Again like VIP, GRP signals through increases in cAMP (Gamble et al. 2007), and GRP application in vivo and in vitro can shift SCN rhythms (Piggins et al. 1995; Antle et al. 2005; Kallingal and Mintz 2006; Gamble et al. 2007). Unlike VIP and VPAC2Rs, blocking GRP receptors (BBR2) does not abolish SCN rhythms, but it does prevent SCN cocultures from restoring rhythms to VIP-deficient SCN (Maywood et al. 2011). Taken together, the data suggest that both VIP and GRP participate in entrainment of SCN circadian rhythms and are important in synchronizing SCN neurons, but that GRP is less powerful in this regard.
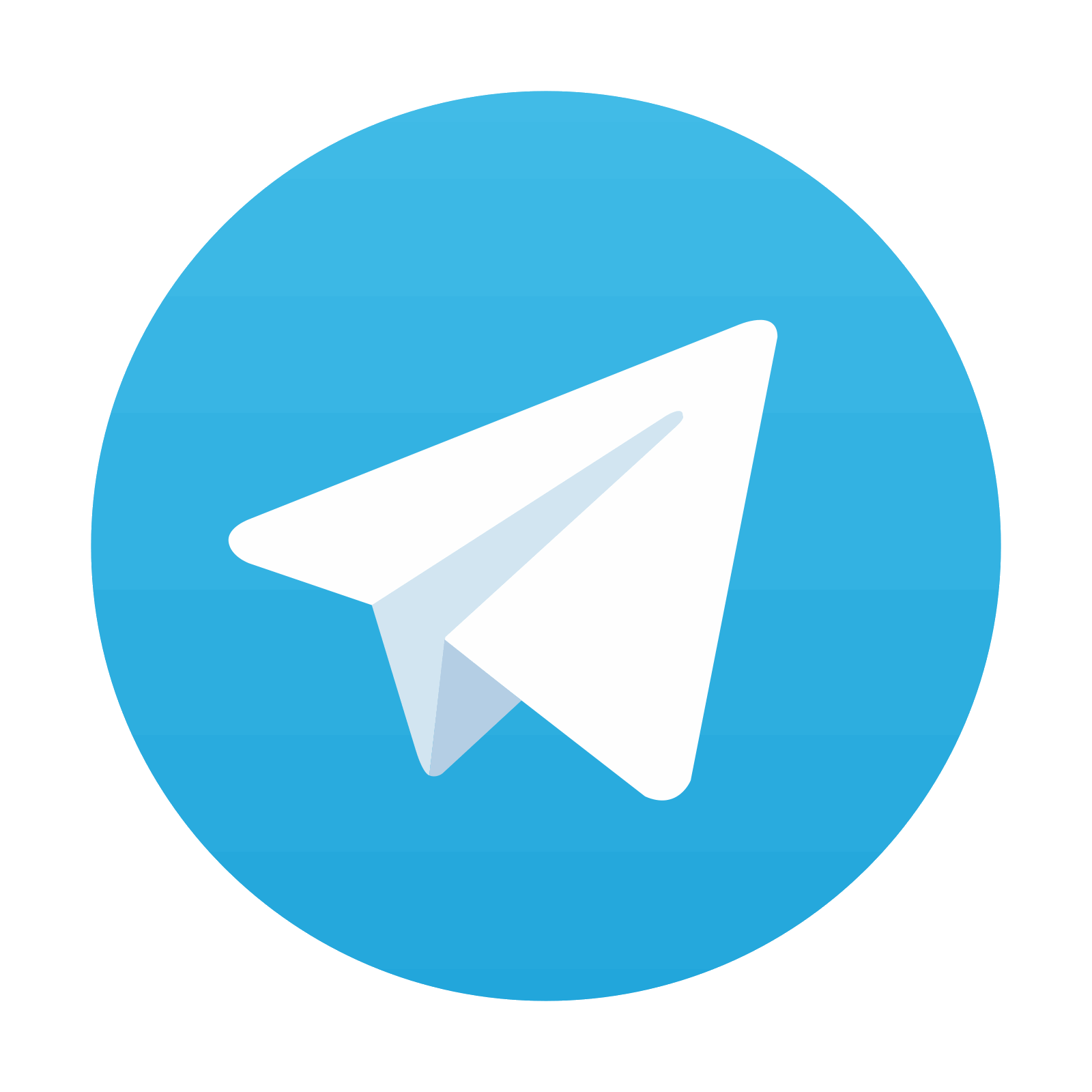
Stay updated, free articles. Join our Telegram channel
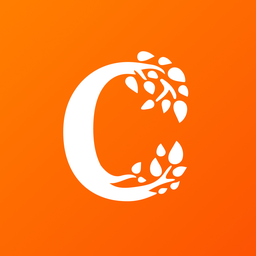
Full access? Get Clinical Tree
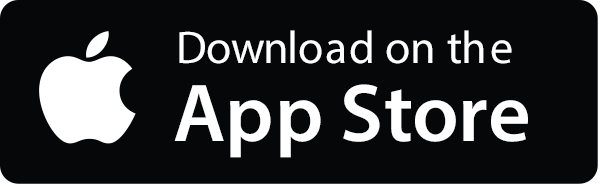
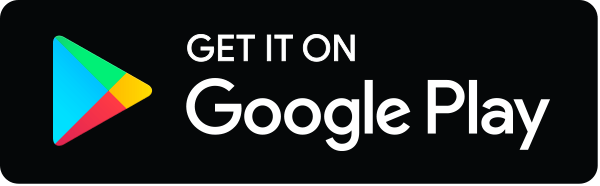