Seth Bleakley CARE Surgery Center, Phoenix, AZ, USA A bone fracture can be defined as a partial or complete break in a bone. Fractures can occur in long or flat bones. Most fractures in healthy bones are due to trauma, where a force is communicated either extrinsically or intrinsically, excessive to the bone’s ability to resist deformation. The type of fracture is dependent on patient age, injury location, and type of inciting force. Patient age affects the composition and mechanical properties of bone. Bone is comprised of collagen rich connective tissue and a mineralized matrix supporting a diverse regenerative cellular population that includes osteoblasts, osteocytes, osteoclasts, and white and red blood cells. Young patients have a higher ratio of collagen rich connective tissue to mineralized matrix, as well as a collagen‐dense periosteum. As such, there may be greater plastic deformation before yield in juvenile fractures; or, in layman’s terms, more bending of bone before it breaks. This has led to the coining of the phrase “greenstick” fracture, where in a juvenile long bone there may be bending or incomplete cortical disruption, or a lack of overlap of fracture ends due to an intact thick periosteum. Mature bone has a high proportion of mineralized cortical bone and, as a result, is more brittle and prone to complete cortical disruption. Another consideration in juvenile fractures is physeal involvement. The physis is defined as a hyaline cartilage plate in the metaphysis of each end of a long bone and is responsible for growth, hence the synonymous term, growth plate. Hyaline cartilage is not mineralized but converted to bone through the process of endochondral ossification. This junction between soft cartilage and hard bone creates mechanical weakness, predisposing the physis to fracture. The incidence of physeal involvement in juvenile fractures is attested by the existence of the classification scheme described by Robert Salter and William Harris in 1963 leading to physeal fractures being known as “Salter‐Harris” fractures1 (see Figure 38.1). Figure 38.1 The five basic fracture types of the Salter‐Harris classification are shown. A S‐H type I fracture is a separation through the physis. A S‐H type II fracture enters the plane of the physis and exits through the metaphysis. A S‐H type III fracture enters the plane of the physis and exits through the epiphysis. A S‐H type IV fracture crosses the physis, extending from the metaphysis to the epiphysis. A S‐H type V fracture is a crushing injury to the entire physis, resulting in the destruction of further growth.1 A S‐H type VI fracture (not shown in this diagram) involves partial crushing of the physis. Injury location and type of inciting force also affect the resultant type of fracture. The diaphysis of mature long bone has a higher proportion of mineralized cortical bone to collagen so is more prone to comminuted fractures than the epiphysis/metaphysis or flat bones. Higher energy extrinsic trauma, such as from vehicular or ballistic impact, results in a higher incidence of bony comminution (e.g., Figure 38.19) than low energy or intrinsic trauma, such as from a fall (e.g., Figures 38.9 and 38.10). Fractures may be described using the following terms: Fracture management has made rapid advances in recent decades largely thanks to a greater understanding of physiology and technological advances in instrumentation. A key contributor has been the AO Foundation (Arbeitsgemeinschaft für Osteosynthesefragen, German for “working group for bone fusion issues”). Founded as a group in Switzerland in 1958 dedicated to improving fracture management, they described what have become known as the AO Principles,2 which have become tenets of clinical decision‐making ever since. The AO principles can be summarized as follows: Anatomical reduction of a fracture will restore bone length and alignment in all three planes. Planes used to describe long bones include the frontal, sagittal, and transverse. Lateral deviation in the frontal plane is known as valgus (Figure 38.2a), while medial deviation is known as varus (Figure 38.2b). Cranial deviation in the sagittal plane is known as recurvatum, while caudal deviation is known as procurvatum (Figure 38.2a). Deviation in the transverse plane is known as torsion and may be internal or external. Bone‐healing with deviation from anatomical origin is known as malunion and can have variable consequences depending on the bone in question. Generally speaking, varus, valgus, and torsional deformities of long bones can have the most significant clinical consequences due to asymmetrical joint‐loading, increased load on ligaments, and gait abnormalities. Therefore, in many cases, diligently restoring skeletal anatomy may offer the best chance of return to normal function. Another major factor in considering anatomical restoration is articular involvement. Fractures involving articular surfaces carry the risk of degenerative joint disease if reduction is not anatomical. A gap in the articular surface will lead to fibrocartilaginous remodeling. A step or irregularity will lead to abnormal joint mechanics, which can wear hyaline cartilage and accelerate osteoarthritis. Consequently, anatomical reduction and rigid fixation is often paramount for favorable long‐term prognosis in fractures involving articular surfaces. An explanation of the need for fracture stability requires a description of strain. Strain has been defined as change in length relative to initial length: Softer tissues tolerate more strain than bone. Soft callus will tolerate 100% strain and still be able to form/heal (e.g., during the first few weeks of fracture healing, this initial soft callus will form despite movement in the fracture zone). Fibrocartilage will tolerate 10–30% strain (e.g., as the soft callus becomes denser, less movement is tolerated). Bone will only form in low strain environments of <2% (e.g., bone will not form until motion is almost eliminated). Strain is concentrated in small fracture gaps. Biologically speaking, unstable fractures heal by producing strain‐tolerant tissues, such as soft callus that eventually mineralizes (becoming visible on radiographs), until enough stability is present to produce strain‐intolerant bone. A fracture gap fills with a hematoma followed by fibrous connective tissue laying down cartilage and osteoid, also known as callus. This connective tissue forms rapidly, and proliferation exceeds the radius of the bone beyond anatomical origins. As the area moment of inertia of a cylinder is directly proportional to the radius to the power of 4 Figure 38.2 Demonstrations of angular limb deformities. (a) CT reconstruction of a canine antebrachium demonstrating lateral deviation in the frontal plane (=valgus) and caudal deviation in the sagittal plane (=procurvatum). (b) Cranio‐caudal radiograph of a canine femur demonstrating medial deviation in the frontal plane (=varus). Source: © Seth Bleakley. If fracture stability can be surgically achieved with a low strain environment <2%, primary bone‐healing can occur without intermediate bone callus.3 This requires very rigid fixation, often coupled with anatomical reduction. Interfragmentary compression is also often required to reduce strain sufficiently for primary bone‐healing. The goal of achieving primary bone‐healing has led to advances in internal fixation instrumentation and techniques. Blood supply is critical to the healing of any tissue including bone.4 Healthy bone requires a substantial blood flow to supply the requisite oxygen and nutrients and to eliminate carbon dioxide, acid, and other metabolic waste products.4 Oxygen plays a critical role in the formation of collagen, the growth of new capillaries, and the control of infection. Sources from which fractures recruit their blood supply include endosteal vessels, periosteal vessels, muscles and surrounding soft tissues. Disruption of these sources can lead to delayed union or nonunion of bone. Successful fracture management must include a careful balance between anatomical reduction, rigid fixation, and preservation of blood supply. There is often a trade‐off among the three. Zealous efforts to achieve anatomical reduction can disrupt the fracture hematoma, strip soft tissues, and reduce vital blood supply. While adding implants may improve fracture stability, each comes at the cost of blocking blood supply, especially where periosteal compression is involved. On the other hand, withholding implants to preserve blood supply can have negative consequences if the construct is not strong enough and subsequently fails. Achieving this balance requires appropriate decision‐making, knowledge of the local anatomy of the soft tissue structures surrounding the fractured bone, and surgical skill for success in treating fractures. That the AO Group started in Davos, Switzerland could be attributed to proximity to Alpine skiing‐related injuries and long bone fractures. Historically, such injuries were managed conservatively with traction and immobilization with casting or limb‐hanging. Disadvantages of such an approach included muscle atrophy, joint stiffness, decreased bone density, and a prolonged rehabilitation period before full function could be (if ever) established. Bone responds to immobilization according to Wolff’s law, which states that it will adapt to the load under which it is placed, or conversely, will become less dense and weaker under decreased load.5 Joint immobilization has been reported to lead to stiffness, replacement of hyaline cartilage with bone, and decreased ligament and tendon strength.6,7 On 17 September 1908, Orville Wright crashed the infamous Wright Flyer and suffered severe injuries that included femoral, pelvic, and rib fractures. His injuries were cared for in the conservative manner of the day at Fort Myer Army Hospital in Arlington, Virginia. He wasn’t discharged until 1 November 1908, almost three months later. His injuries reportedly never fully healed, he suffered pain in his pelvis and ribs for the rest of his life, and his time as a pilot was subsequently greatly reduced.8 Some of the most obvious benefits of modern fracture management include short hospitalization time, early mobilization, and early return to function. Early mobilization is both prerequisite to, and a benefit of, successful fracture management. Successful fracture management must include a careful balance between anatomical reduction, rigid fixation, and preservation of blood supply. A bias in this balance has been described paradigmatically as the “Carpenter” versus the “Gardener” approach. Simplistically, the “Carpenter” will be more concerned with anatomical reduction and the appearance of the repair visually and radiographically. This will be reflected in the invasiveness of the approach and the number of implants necessary to achieve such, often with bone plates, lag screws, and cerclage wires applied with the goal of interfragmentary compression. This can offer many of the aforementioned benefits, including restoration of anatomy/alignment, rigid fixation, early return to function, and the potential for primary bone‐healing. However, there is often a biological cost. Draining the fracture hematoma through open reduction and internal fixation has been shown to delay healing.9 In experimentally‐induced femoral fractures in rats, drainage of the fracture hematoma led to approximately 50% bending rigidity of the fracture callus at four‐weeks post‐fracture compared to the intact hematoma controls.9 The effect was even more detrimental when drainage of the hematoma was delayed by two or four days, with intact hematoma fractures being 5–10 times stronger at four weeks.9 The intact hematoma has been shown to provide growth factors and mesenchymal cells vital to rapid angiogenesis and fibrocartilaginous callus. Application of implants directly to bone involves both stripping surrounding soft tissues, such as muscle, and compressing periosteal vasculature. The result is reduced blood supply to the bone for maintenance and healing. Cortical bone beneath compressed implants becomes devitalized,10 and bone‐healing may be delayed or impaired. Delayed bone‐healing will increase the amount of time and subsequent cyclic‐loading forces on the construct and implants. This can increase the incidence of screw loosening, material fatigue, and implant failure. Ironically, a stronger, more rigid construct can have a higher incidence of failure if there is a threshold detriment to the biological component of fracture healing. If the application of implants robs the fracture zone of its ability to heal quickly, the construct will have to resist fracture forces longer and may fail. Simplistically, the “Gardener” will be more concerned with the preservation of soft tissues and blood supply than anatomical reduction and rigid fixation. Surgical approaches will be less invasive than those of the “carpenter,” and, therefore, less disruptive to soft tissues and periosteum. Implants will be more minimalistic and more focused on spanning the fracture zone rather than reconstructing it. Fragments and interfragmentary compression will often be ignored, with the assumption that the body will integrate and remodel fragments through biological processes. Biological osteosynthesis will occur more rapidly due to the preservation of fracture hematoma and soft tissues providing blood supply. There are both mechanical and biomechanical costs to bias toward the “Gardener” approach. Implants distant to the axis of the bone will reduce the stiffness of the construct. A minimalistic blood supply‐friendly construct may have reduced load‐sharing with bone, be less mechanically adequate, and may create a risk of early failure. Since primary bone‐healing requires a low‐strain environment, a repair without rigid fixation and interfragmentary compression can only result in secondary bone‐healing with intermediate callus. Excessive micromotion may even lead to delayed or nonunion. Lack of attention to anatomical reduction can lead to malunion and angular deformities with subsequent clinical consequences. Table 38.1 Summary of clinical data related to the treatment of human femoral fractures over three decades. Source: Adapted from Rozbruch et al. 11 As surgical techniques evolved toward biological osteosynthesis, complication, and revision rates significantly decreased, while bone‐healing time and success rates significantly improved. As one would expect, neither paradigm is superior, and successful fracture management necessitates a careful balance between the “Carpenter” and “Gardener” approaches. Every fracture must be critically evaluated, taking mechanical, biological, and patient and client factors into account before making a treatment decision. Nevertheless, there has been a historical trending toward more minimally invasive fracture repair to promote biological osteosynthesis with data showing an improved clinical union success rate.11 As demonstrated in Table 38.1, over a period of ~30 years, a trend toward reducing plate holes, increasing plate length, and decreasing screw number and density, there has been a decrease in implant failures, delayed unions, malunions, and surgical revisions. While there may be a list of variables affecting these outcomes, repairs that better preserve biological osteosynthesis have an improved prognosis. Discussing fracture repair options first necessitates an understanding of fracture forces. Repair choice will affect which forces are resisted and is a factor of both fracture configuration and the mechanical competencies of each device or treatment option. Forces relevant to fractures include bending, shear, tension, compression, and rotation (Figure 38.3).
38
Principles of Fracture Repair
Introduction to Fractures
General Principles and Physiology of Fractures
Restoration of Anatomy
Establishment of Stability
where I is area moment of inertia, ro is the outer diameter, and ri is the inner diameter), a small increase in radius creates a large increase in resistance to bending, and, therefore, rapid increase in stability. This lowers the strain, allowing formation of osteoid and bony remodeling until normal cortical bone can be formed, a process known as Haversian remodeling.3 This entire process is known as secondary bone‐healing and is the mechanism by which unstable fractures heal. However, if fracture instability is such that strain remains excessive for callus formation and biological stabilization to ever materialize, a nonunion will occur.
Preservation of Blood Supply
Early Mobilization
Minimally Invasive Osteosynthesis and the Carpenter Versus Gardener Paradigm
Variable
Group 1 (1970s)
Group 2 (1980s)
Group 3 (1990s)
Number of plate holes
12
14
15
Plate length (cm)
22
26
28
Number of plate screws
11
11
7
Plate screw density
95%
84%
45%
Primary bone graft
16%
30%
4%
Implant failures
19%
10%
4%
Delayed unions
14%
7%
0
Nonunions
10%
3%
4%
Malunions
10%
7%
0
Re‐operations
43%
31%
13%
Clinical union (months)
5
5
3
Success rate
62%
83%
87%
Fracture Forces
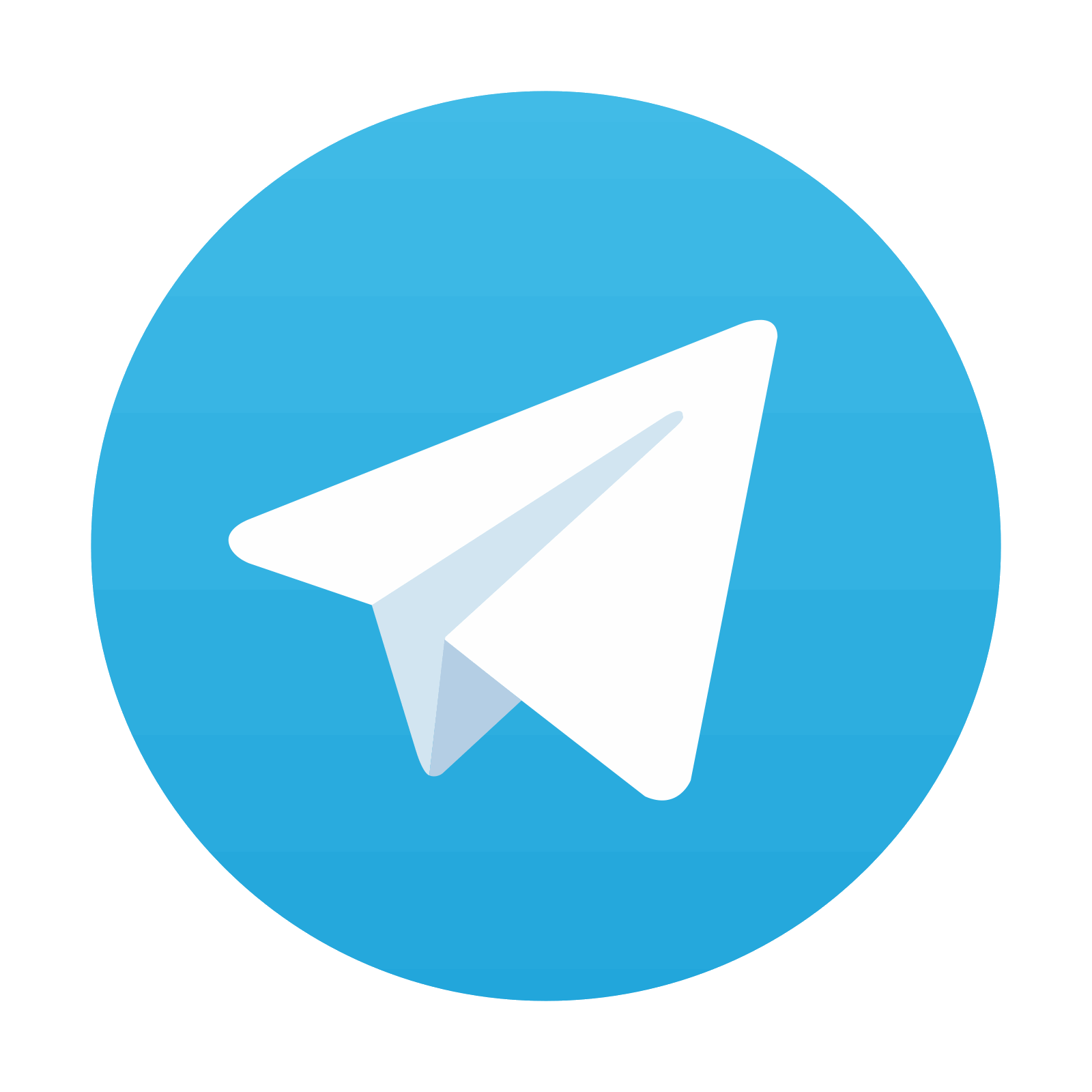
Stay updated, free articles. Join our Telegram channel
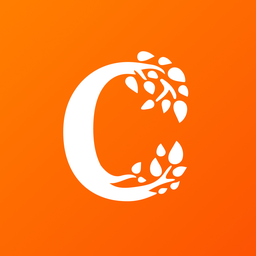
Full access? Get Clinical Tree
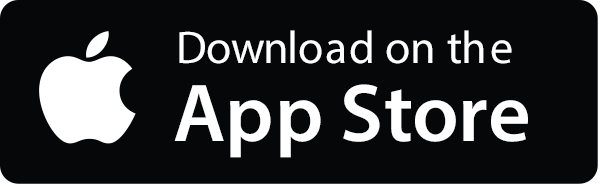
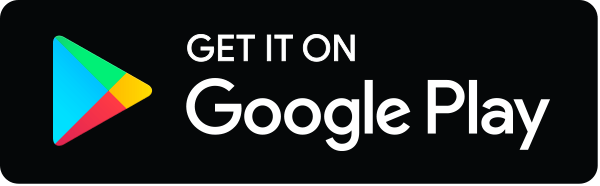