Stuart C. Clark‐Price1 and Julie R. Fischer2 1 Department of Clinical Sciences, College of Veterinary Medicine, Auburn University, Auburn, Alabama, USA 2 Veterinary Specialty Hospital of North County (Ethos Veterinary Health), San Marcos, California, USA The kidneys receive and require 20–25% of cardiac output to appropriately perform their intricate and multifaceted role in the maintenance of physiologic homeostasis. By its nature, general anesthesia alters hemodynamics and organ perfusion, thus inherently posing specific potential risk to healthy kidneys and even more so to diseased or compromised kidneys. Renal disease and functional impairment occur frequently in veterinary patients, particularly in geriatric ones, with a 1–3% overall prevalence in cats, up to 20% of cats having chronic kidney disease (CKD) during their lifetime, and 35–80% of cats older than 15 years affected [1–3]. The prevalence of CKD in dogs is lower and has been reported at 0.05–3.74% depending on inclusion criteria, with the largest and most recent study reporting a 0.21–0.37% overall prevalence [4]. Histopathologic examination of the kidneys of nonazotemic cats shows chronic degenerative changes including glomerulosclerosis, tubular atrophy, interstitial inflammation, and fibrosis that increases in severity with age [5]. Similar chronic changes have been shown in aged canine kidneys as well, suggesting that geriatric patients may be at heightened risk of renal damage from nephrotoxic drugs and hemodynamic shifts, even when these patients are nonazotemic [6]. Equine and ruminant patients have a much lower overall prevalence of renal disease than cats and will be discussed less in this chapter. The overall prevalence of CKD in horses is 0.12% with a prevalence of 0.23% in horses older than 15 years of age, increasing to 0.51% in stallions older than 15 years of age [7]. Acute kidney injury occurs more commonly than CKD in horses, with 23% of horses presenting for colic having evidence of injury and as many as 25% of neonatal foals presenting for intensive care [8]. Because animals with renal disease will routinely require anesthesia, clinicians must have a working knowledge of renal physiology and pathophysiology, as well as a thorough understanding of both the potential renal effects of the sedative, analgesic, and anesthetic drugs they select and how best to protect kidney health and function during their use. A complete in‐depth overview of the anatomy and physiology of the renal system is beyond the scope of this chapter; the reader is referred to medical anatomy and physiology textbooks for further detailed descriptions [9–11]. Grossly, the urinary system can be divided into the kidneys, the ureters, the urinary bladder, and the urethra. The kidney can be further divided into the cortex, medulla, and renal pelvis. The renal pelvis is essentially the expanded proximal portion of the ureters, which carry urine from the kidney to the urinary bladder. Between species, there are several anatomic differences that exist within the kidney. Dogs, cats, horses, and small ruminants have unilobar kidneys that contain a renal crest or basin within the renal pelvis that collects urine and empties into the proximal urethra. Additionally, gland‐like structures in the wall of the horse’s renal pelvis secrete a mucous‐like substance that is responsible for the cloudy and foamy nature of normal horse urine. Swine and bovine kidneys are multilobar kidneys that contain renal papillae (medullary extensions or pyramids) that empty into cup‐like calyces that then empty into the renal pelvis. The gross shape of the kidney is fairly similar in that most species have the classic kidney shape with a smooth surface and two poles. The exceptions are the right kidney of the horse, which tends to have a heart‐like shape, and the lobulated kidneys of the bovine species, due to incomplete fusion of the kidney lobes. The ureters are smooth muscle‐lined tubes that carry urine from the kidney to the bladder. The urinary bladder can be anatomically divided into the body and the neck or trigone region. The body consists of a compliant three‐layered muscular wall that can accommodate incoming urine for storage and later voiding. This allows the bladder to vary greatly in shape, size, and position within the pelvis and abdomen. The urethra is a muscular tube that connects the bladder to the genitals, allowing transit of urine from the bladder to the external environment. Anatomic variations of the urethra exist based on gender and species. The functional units of the kidneys are nephrons (Fig. 43.1), which consist of a renal corpuscle and associated tubule, span the cortex and medulla of the kidney, and exist within a vital milieu called the renal interstitium. The renal interstitium is composed of cellular components (“interstitial cells”) residing in a loose glycosaminoglycan matrix. The majority of interstitial cells are specialized fibroblasts that structurally stabilize the kidney by weaving around and between nephrons, and by connecting to each other via intermediate‐like junctions. These fibroblasts have very well developed rough endoplasmic reticulum, ribosomes, and Golgi apparatus to support their many synthetic functions. A smaller population of marrow‐derived cells migrates through the interstitium; in healthy kidneys, most of these are major histocompatibility complex (MHC) class II‐expressing dendritic cells, but in disease states, particularly inflammatory states, invasion by other mononuclear cells (e.g., lymphocytes, monocytes/macrophages, and other dendritic cells) is common. The interstitial matrix is a living interconnection among the three chief functional components of the kidney, that is, the vasculature, the tubules, and the glomeruli, and serves as a thoroughfare between the renal tubules and the peritubular capillaries. Solute and water transport occurs from the tubular lumina through the interstitium and into the peritubular capillaries, while oxygen and nutrients travel from the capillaries through the interstitium to nourish the tubular cells [12]. Figure 43.1 Anatomy and physiologic function of each portion of the nephron. Source: DiBartola [11], reproduced with permission of Elsevier. Renal tubules are responsible for modification of plasma filtrate into urine and are described in functional segments termed the proximal tubule, loop of Henle, distal tubule, and collecting duct. Tubules comprise the substantial majority of renal mass, with blood vessels, interstitium, and renal corpuscles present in much smaller quantities. The cortex contains the corpuscles, which are tight, spherical convolutions of glomerular capillaries surrounded by a specialized proximal tubular outpouching called Bowman’s capsule. Initial filtration of plasma occurs at the glomerulus across the glomerular filtration barrier (described later in the chapter); the filtrate then flows into Bowman’s capsule and from there into the proximal renal tubule. The cortical parenchyma consists of renal tubules and capillaries packed around glomeruli in seemingly random fashion, surrounded by small amounts of intercalated interstitium. The medulla consists entirely of tubules, arterioles, capillaries, and interstitium, packed tightly together with the tubules and vessel networks arranged radially in near‐parallel formation within the parenchyma and in much more organized fashion than in the cortex. The final tubular product, urine, flows into the renal pelvis in transit to the ureter, which then propels the urine by peristaltic waves into the bladder [13]. The kidneys govern or play a significant role in a myriad of homeostatic processes, chiefly maintaining water and electrolyte balance, acid–base regulation, systemic blood pressure management, extracellular fluid volume composition, excretion of dissolved foreign substances and metabolic wastes, vitamin D production and calcium–phosphorus balance, hormone secretion, metabolism and excretion, erythrocyte production, and even gluconeogenesis. They accomplish many of these functions through generation of urine by the processes of filtration, reabsorption, and secretion and receive about 25% of the cardiac output in order to do so. In fact, the renal arteries branch directly from the caudal aorta, providing the high percentage of cardiac output necessary to facilitate filtration and maintain the kidneys’ high metabolic activity (i.e., very high oxygen and substrate consumption). Kidneys, especially the proximal tubules, are particularly susceptible to hypoxic damage and even short‐term renal ischemia can lead to acute kidney injury (AKI). Renal blood flow (RBF) is regulated by extrinsic nervous and hormonal control and by intrinsic autoregulation. The renal vasculature is highly innervated by sympathetic constrictor fibers originating in the spinal cord segments between T4 and L1, yet the kidneys lack sympathetic vasodilating fibers and parasympathetic innervation. Dopamine receptors in the renal vasculature and tubules help regulate vasodilation and blood flow. There are two known subtypes of dopamine receptors, D1 and D2. Both of these receptors have been identified in dogs, rats, rabbits, and other animals. It was thought that cats did not possess renal dopamine receptors; however, in 2003, a D1‐like receptor was identified that is considered to be different from receptors found in rats, dogs, or humans [14]. Intrinsic autoregulation of RBF is demonstrated by a constant flow when the mean arterial blood pressure ranges from 80 to 180 mmHg. When the mean arterial blood pressure is in this range, the kidney can control blood flow via alteration of resistance in the glomerular afferent arterioles. Although the exact mechanism of renal autoregulation is not known, the phenomenon protects glomerular capillaries during hypertension and preserves renal function during hypotension. In addition to renal autoregulation, extrinsic forces (e.g., neural, hormonal, and pharmacologic) and intrinsic forces (e.g., kidney disease) may cause alterations in RBF. Catecholamines are major hormonal regulators of RBF. Epinephrine and norepinephrine cause dose‐dependent changes in RBF. Low doses increase arterial blood pressure and increase RBF through increased cardiac output, whereas higher doses cause a decreased RBF through increased vascular resistance. The renin–angiotensin–aldosterone system, discussed later in this chapter, is also an important regulator of RBF. In addition, prostaglandins play an important role in the regulation of RBF. Prostaglandin‐E2 (PGE2) and prostaglandin‐I2 (PGI2 or prostacyclin) cause vasodilation within the kidney. Generation of PGE2 and PGI2 occurs through the upregulation of cyclo‐oxygenase enzymes; in particular, the cyclo‐oxygenase‐2 (COX‐2) enzyme plays a largely constitutive and protective role. The COX‐2 enzyme is found mainly in the macula densa but can also be found in other areas of the cortex and the medulla. During low blood flow or hypotensive states, COX‐2‐derived prostaglandins promote natriuresis, renin release, and vasodilation of afferent arterioles to preserve RBF. Although the kidney receives a high percentage of the cardiac output, blood flow is not evenly distributed throughout the kidney. The renal cortex receives the majority of the blood (90–95%), thus leaving the medulla relatively hypoperfused and hypoxic. This dichotomous blood flow strategy maximizes flow‐dependent activities in areas of the kidney that specialize in high‐efficiency filtration. Hydrostatic pressure drives plasma filtration across the glomerular filtration barrier and into the proximal convoluted tubules. The filtration barrier consists of the glomerular capillaries, the glomerular basement membrane, and specialized epithelial cells called “podocytes.” The glomerular capillaries consist of a single layer of endothelial cells with small fenestrations that provide the filtration surface and allow only fluid, very small proteins, and electrolytes to be filtered. Under these cells is the glomerular basement membrane, an acellular, trilaminar structure composed of various proteins and proteoglycans, that provides a scaffold for the epithelial cells and a negatively charged barrier that excludes larger negatively charged molecules (chiefly proteins). The last layer of the glomerular filtration barrier is formed by the network of extensively branching podocytes that cover the entire glomerular surface. The tiny spaces between the interdigitating processes of these cells are specialized junctions called “slit pores” or “slit diaphragms,” which provide the most size‐restrictive portion of the filtration barrier. With the exception of albumin and larger proteins, the glomerular filtrate received by Bowman’s capsule is very similar in composition to plasma (Table 43.1). The rate of formation of the filtrate, or glomerular filtration rate (GFR), is a measurable parameter that can be evaluated clinically to determine renal excretory function. GFR is expressed as milliliters of glomerular filtrate per kilogram of body weight formed per minute (mL/kg/min). Blood flow into the glomeruli for filtration is under pressure and is regulated by afferent (preglomerular) and efferent (postglomerular) arterioles. The amount of filtrate formed is directly related to the pressure across the capillaries, and this pressure can be described mathematically using Starling’s equation: where Q is the net fluid movement across the capillaries, Kf is the filtration coefficient (which depends on the permeability and length of the filtration surface), Pc is the capillary hydrostatic pressure, Pi is the interstitial hydrostatic pressure, σ is the reflection or filtration coefficient, πc is the capillary oncotic pressure, and πi is the interstitial oncotic pressure. When measured at the afferent arteriole end of the glomerulus, the net filtration pressure is about 10 mmHg, resulting in a net outflow. When measured at the efferent arteriole end of the glomerulus, the net filtration pressure is about 0 mmHg, resulting in zero net outflow. Table 43.1 Major activity and filtrate composition in the nephron. RBF and, thus, usually GFR are maintained within a consistent range in spite of changes in systemic blood pressure by an autoregulation system controlled in large part by the renin–angiotensin–aldosterone hormone system (RAAS). When blood pressure and subsequent renal perfusion decrease, the enzyme renin is released from specialized juxtaglomerular cells located in the walls of the afferent arterioles, just proximal to the glomeruli. Renin acts on angiotensinogen (produced by the liver) to release angiotensin I, which is swiftly converted to angiotensin II (AT‐II, a potent vasoconstrictor) by angiotensin‐converting enzyme (ACE). This conversion occurs chiefly while blood flows through the small vessels in the lungs, since most ACE is produced by the pulmonary small vascular endothelium. The kidneys and other blood vessels also produce some ACE and generate a smaller amount of AT‐II locally. AT‐II directly increases blood pressure through constriction of smooth muscle in arterioles but also has multiple endocrine and paracrine effects. Locally within the kidney, AT‐II activates sodium uptake in the tubules of the nephron, promoting fluid retention and increased blood volume. At the adrenal gland, AT‐II increases release of the steroid hormone aldosterone, which promotes sodium conservation, potassium elimination, and increased fluid retention and blood pressure. At the pituitary gland, AT‐II enhances vasopressin (also known as antidiuretic hormone) release, which increases water reabsorption in the kidneys through the insertion of water channels (aquaporin‐2) into the membrane of the distal tubules of the nephron. Vasopressin also has direct vasoconstrictive properties through G‐protein‐coupled V receptors in vascular endothelium. Additionally, AT‐II induces intrarenal release of the vasodilating prostaglandins PGE2 and prostacyclin (PGI2) that counteract the vasoconstricting effects of AT‐II, thereby preventing excessive intrarenal vascular resistance and local ischemia. A second intrinsic system, called “tubuloglomerular feedback,” also contributes to the autoregulatory system that helps maintain GFR. A distinct group of epithelial cells within the distal convoluted tubule (DCT), the macula densa, contact the glomerulus between the afferent and efferent arterioles as part of the juxtaglomerular apparatus (JGA). Osmoreceptors within the macula densa cells sense decreased sodium concentrations within the tubular lumen and initiate a cascade of events resulting in renin release from the juxtaglomerular cells. Alternatively, increased sodium chloride concentrations result in an undefined cascade of events that suppresses renin release and leads to production of vasoactive factors (nitric oxide, adenosine triphosphate, prostaglandins) that reduce GFR and promote free water conservation. Filtered fluid that leaves the glomerulus next enters the proximal convoluted tubules (PCTs). The major function of the PCT is to reabsorb the majority of the filtrate; in fact, more than 60% of the filtered substances are reabsorbed in the PCT. Transport across the PCT membrane into the interstitial tissues and then into the vascular space occurs via both active and passive transport with Starling’s forces dictating the passive phases. The majority of ions filtered at the glomerulus (Na+, K+, Ca2+, Cl–, and HCO3–) are reabsorbed in the PCT so that filtrate leaving the PCT and entering the loop of Henle has lower levels of electrolytes than plasma. Water passively follows the active reabsorption of electrolytes so that the osmolality of the tubular fluid is similar to that of plasma at both ends of the PCT. Organic low‐molecular‐weight proteins (insulin, glucagon, parathyroid hormone, etc.) that were filtered are also actively reabsorbed in the PCT. Secretion of substances also occurs in the PCT. Organic ionic wastes are eliminated via secretion into the filtrate; these include protein‐bound exogenous substances that are not filtered at the glomerulus, for example, endotoxins, antibiotics, and anesthetic and analgesic drugs such as morphine and ketamine, and endogenous wastes such as bile salts, urates, and prostaglandins. PCT secretion is exceptionally important for endogenous waste removal in birds and reptiles. In mammals, one of the main waste products from muscle metabolism is urea, which is freely filtered at the glomerulus; however, uric acid is the waste product from muscle metabolism in birds and reptiles. Uric acid is not filtered at the glomeruli, and its removal is dependent upon active mechanisms in the PCT in these species. Next, the remaining filtrate enters the descending loop of Henle (DloH). Metabolic activity within the DloH is minimal, and little to no active transport process occurs. Pure water reabsorption and minimal solute drag occur as the filtrate travels through the DloH to the highly active ascending (AloH) or thick portion of the loop of Henle. The AloH is one of the most metabolically active parts of the tubule and the entire kidney. It is composed of simple cuboidal epithelium with many mitochondria, which provide the energy for high‐capacity active transport. Here, the filtrate is further modified as the majority of electrolyte reabsorption within the loop of Henle takes place in the AloH. An abundant concentration of Na+‐K+ ATPase pumps can be found on the luminal surface of the tubules, which are responsible for the active transport of Na+, K+, and Cl− into the cell and out of the filtrate. Other ions such as Mg2+ and Ca2+ move down a cation‐selective paracellular pathway into the interstitium. In the case of K+ in particular, hyperkalemia can result in electrical conduction pathology, and therefore, both apical and basolateral K+ channels exist to increase secretion into the filtrate for eventual removal in the urine. As the AloH is highly metabolically active, it has a high demand for substrates such as oxygen and, therefore, has increased sensitivity to damage during hypoxemia or hypotension. Additionally, several medications can exert their effects both therapeutically and pathologically at the AloH. For example, furosemide works as a diuretic by inhibiting Na+‐K+ ATPase pumps and preventing electrolyte and fluid reabsorption while aminoglycoside antibiotics such as gentamicin can inhibit protein synthesis and result in acute tubular necrosis in the PCT and the AloH. The AloH is impermeable to water so that filtrate entering the DCT is hypotonic; this is, in fact, how the kidney forms dilute urine in the absence of antidiuretic hormone (ADH). After the AloH, the highly modified hypotonic filtrate enters the DCTs. Electrolyte and water reabsorption occurs to modify the filtrate further; however, K+ and H+ secretion is of major importance in the DCT. Because the majority of HCO3− was reabsorbed in the PCT, only a very small amount of HCO3− remains, and therefore, both K+ and H+ must be actively pumped into the lumen to counter an imbalance between negatively and positively charged ions. This can result in K+ and H+ competing with each other for secretion and is often the reason why hyperkalemia is associated with acidemia. Finally, the filtrate enters the collecting duct where it can be modified one more time before being classified as urine. Collecting duct tubular cells must be impermeable to water when patients are overhydrated and permeable to H2O during dehydration. This is accomplished via the actions of the hormone vasopressin (ADH). When states of dehydration exist, vasopressin is released from the neurohypophysis, stimulated by increased plasma osmolality, and its presence at the level of the kidneys induces the translocation and insertion of water channels (aquaporin‐2) into the cells of the DCT and collecting duct. Water is then allowed to be reabsorbed, resulting in a more concentrated urine. Interestingly, α2‐adrenergic receptor agonist drugs, such as xylazine, block the vasopressin receptors located within the collecting ducts, preventing water reabsorption. This can be observed clinically as animals voiding large amounts of dilute urine after sedation with an α2‐adrenergic receptor agonist and can result in worsening dehydration in animals with a negative fluid balance. On leaving the collecting duct, the filtrate is considered to be urine, where it is gathered in the renal pelvis and transported to the urinary bladder via the ureters for storage and voiding. Any determination of intrinsic renal dysfunction must start by identifying/excluding and/or correcting any prerenal (i.e., anything that decreases RBF) and postrenal (i.e., anything that blocks normal transit of urine from the kidney to the urethral orifice) causes of azotemia. Evaluation of blood urea nitrogen (BUN) and serum creatinine concentrations in combination with urine specific gravity has long served as the chief method of routine clinical diagnosis and serial monitoring of renal function in dogs and cats. As GFR declines, serum creatinine increases (though in nonlinear fashion) and, since it is affected by fewer extrarenal factors than BUN, serum creatinine measurement is the most commonly employed indirect estimate of GFR. Unfortunately, neither BUN nor serum creatinine is particularly sensitive or specific for intrinsic renal disease and, at best, provides a rough index of GFR [15–19]. Decreased diagnostic accuracy for these analytes results in part because BUN and serum creatinine generally do not rise out of the reference range before roughly 75% of functional renal mass has been lost; however, many other factors influence this as well. In dogs and cats with CKD, compensatory hypertrophy and hyperfiltration in the remaining functional nephrons can also mask the degree of degeneration present. The relationship between GFR and the inverse of serum creatinine concentration is logarithmic rather than linear and can, therefore, lead to overestimation of renal function in early CKD and underestimation in later‐stage disease. Because creatinine is a breakdown product of creatine phosphate generated during normal myocyte metabolism, serum creatinine concentration can vary greatly relative to an individual animal’s muscle mass. Heavily muscled dogs, such as Greyhounds, may have serum creatinine measurements that fall above the reference range in spite of normal kidney function, while sarcopenic animals often have serum creatinine measurements that significantly under‐represent the severity of kidney disease present. Total ingested protein load, among other extrarenal factors, can markedly increase BUN concentration (and to a lesser extent, serum creatinine), while hepatic insufficiency or a very low protein diet may decrease it, irrespective of kidney function. The limitations of BUN and serum creatinine in assessing renal function have led to investigation of other biomarkers for this purpose, one of which is symmetric dimethylarginine (SDMA) [20,21]. This molecule is an endogenous product of intracellular protein methylation and degradation that is chiefly renally excreted and freely filtered at the glomerulus. Serum concentration of SDMA has been shown by clearance studies in azotemic and nonazotemic cats [17] and in dogs with hereditary nephropathy [18] to be strongly inversely correlated with GFR (in linear fashion). Serum SDMA concentration increases when approximately 40% of functional renal mass is lost, making it a more sensitive marker of early CKD in dogs and cats than serum creatinine, which increases after approximately 75% functional decrement, as mentioned above. Unlike serum creatinine, SDMA is not affected by increased or decreased muscle mass in a given animal, and studies specifically examining non‐renal modifiers of SDMA in dogs have shown that asymptomatic mitral regurgitation, age, breed, sex, and vigorous exercise have no influence on serum SDMA concentrations [22,23]. On average, monitoring of SDMA permits detection of CKD 17 months earlier in cats and 9 months earlier in dogs than does assessment of serum creatinine [19,21,24,25]. As with BUN and creatinine, any process that results in decreased GFR can increase SDMA concentration; thus, although it will also be elevated in animals with decreased GFR due to AKI, SDMA concentration cannot be used to distinguish between AKI and CKD [26]. Currently, the International Renal Interest Society (IRIS) recommends using both serum creatinine and SDMA as complementary parameters in the staging of CKD. Regardless of which biochemical parameters are used, their longitudinal/serial monitoring in the same patient will improve detection of declining renal function, even if values remain within normal limits. For example, a serum creatinine concentration that increases from 0.6 to 1.2 mg/dL over time may indicate a ≥ 50% reduction in renal function (assuming that muscle mass has not changed, and hydration status is normal). Since normal kidneys regulate total body water by adjusting the amount excreted in urine, measurement of urine specific gravity (USG, comparison of the weight of a solution to the weight of water) can help assess renal tubular function; however, like BUN and serum creatinine, USG is neither a sensitive nor specific measure of function. The normal dog kidney can produce a USG > 1.030, while a normal feline kidney can produce a USG of > 1.035, depending on water balance at any given time, though a physiologically appropriate USG can vary from 1.001 to up to 1.075 in the dog and 1.085 in the cat. When renal function declines beyond a certain point, the kidneys lose the capacity to concentrate and dilute filtrate; thus, the urine produced remains in a fixed range called the isosthenuric range (1.008–1.012), which is the USG range of unmodified glomerular filtrate entering the proximal tubule. In dogs, decrements in urine‐concentrating ability are not detectable until approximately two‐thirds of the nephrons are non‐functional. In cats, this proportion is thought to be higher, since it is not uncommon for cats with renal azotemia (and thus more than 75% loss of renal function) to retain the ability to produce significantly hypersthenuric urine. In addition to this lack of sensitivity, extrarenal influences can compromise urine‐concentrating ability, as seen with decreased ADH secretion or responsiveness (e.g., hypercalcemia, hyperadrenocorticism, glucocorticoid exposure, etc.), use of diuretic medications, renal medullary washout, significant glucosuria, and other causes. Hypovolemia and consequent decreased GFR in these patients can thus result in azotemia with inappropriate isosthenuria, in spite of underlying normal renal functional capacity [27]. As an alternative or complement to standard clinicopathologic tests, the detection and quantification of multiple urine biomarkers, including clusterin, cystatin C, γ‐glutamyl transpeptidase (GGT), N‐acetylglucosaminidase (NAG), and neutrophil gelatinase‐associated lipocalin (NGAL), have been proven able to indicate pre‐azotemic nephron damage in the dog [28–31]. These biomarkers may be useful both in the acute (NAG, GGT, NGAL, cystatin C, and clusterin), and chronic (cystatin C, NGAL, and SDMA) setting [29,32,33]. Since some enzymes originate from specific cellular organelles, those enzymurias mark damage to a specific anatomic site. For example, γ‐glutamyl transpeptidase (GGT) originates from the proximal tubular brush border and N‐acetylglucosaminidase (NAG) is a lysosomal enzyme. Enzymuria usually precedes azotemia and decreased urine‐concentrating ability associated with nephrotoxic proximal tubular injury by several days. Urine GGT:creatinine and NAG:creatinine ratios have been shown to reflect accurately 24‐h urine GGT and NAG excretion in dogs, if determined before the onset of azotemia [28]. Determination of baseline urine GGT:creatinine and NAG:creatinine ratios, therefore, could be considered in dogs with possible pre‐existing acute renal insult that are to receive potentially nephrotoxic drugs. Two‐ to threefold increases in the GGT:creatinine or NAG:creatinine ratio over the baseline are suggestive of clinically relevant tubular damage. Drug therapy should be discontinued if this occurs. The repeatable presence of renal proteinuria is both a marker of renal disease and also a negative prognostic factor when identified in animals with CKD [34–37]. Detection of greater than normal amounts of protein in the urine, usually initially by dipstick analysis, should prompt classification by identification of origin: physiologic (e.g., from fever, extreme heat/cold, and seizure), prerenal (e.g., due to hyperglobulinemia), postrenal (e.g., from genital or lower urinary tract inflammation), or renal, due to intrinsic tubular or glomerular disease. Significant proteinuria of renal origin is persistent (multiple assessments above reference range, separated by 7–14 days) and associated with a normal/inactive urine sediment. Urine protein concentration is quantitated in a ratio with the concentration of creatinine (urine protein:creatinine, UPC); a UPC > 0.4 is considered abnormal in the cat and a UPC > 0.5 is considered abnormal in the dog. Renal proteinuria can be associated with increased filtration through damaged glomerular capillary walls (i.e., glomerular proteinuria, UPC usually > 2.0) and/or decreased reabsorption by tubular epithelial cells (i.e., tubular proteinuria, UPC usually < 2.0). Tubular lesions in patients with AKI may result in proteinuria; however, most often this is a transient finding directly related to tubular epithelial damage and resolves with regeneration of the tubular epithelium if the patient recovers. When renal proteinuria is seen in patients with CKD, it is generally a chronic finding. The sensitivity of renal proteinuria as a marker of CKD is high, especially if the UPC or species‐specific albuminuria is serially monitored [35,36,38–40]. Documenting persistent renal proteinuria with a UPC > 2.0 often signals the presence of glomerular disease, full discussion of which is beyond the scope of this chapter, but renal proteinuria of any magnitude above reference range can induce ongoing tubulointerstitial inflammation and fibrosis, nephron loss, and accelerated progression of CKD [36,40,41]. The variety of mechanisms by which this happens are not fully understood, but proximal renal tubular overload/damage from high‐protein filtrate and the resulting ongoing interstitial inflammation, renal oxidative stress, and hypoxic renal environment are among them [42]. Patients with significant renal proteinuria, even nonazotemic ones, should thus be considered likely more vulnerable to renal damage from nephrotoxic substances and abnormal hemodynamic states than similar nonproteinuric patients would be. Renal clearance is the rate at which a substance is completely cleared from a specified volume of plasma. Substances used to measure renal clearance must be freely filtered by the glomerulus (not protein bound) and not be affected by tubular reabsorption or secretion, or by metabolism elsewhere in the body. In addition, the substance used must not alter renal function. Measurement of GFR by clearance studies, generally accepted as the most sensitive and specific method to assess renal function, may be performed to achieve greater diagnostic accuracy than routine laboratory testing provides. This process can be particularly useful when renal disease is suspected prior to the onset of renal azotemia. Though GFR measurement using plasma clearance of creatinine or iohexol, and also via technetium‐based renal scintigraphy, has been validated in dogs and cats and is described below, these procedures are significantly more cumbersome, time consuming, and expensive than standard blood and urine testing, plus availability of nuclear medicine is particularly limited. For those reasons, clearance studies are not commonly performed for routine assessment of renal function, even at specialty centers. Specific, detailed instructions for clinical GFR assessment via iohexol or creatinine clearance can be found on the IRIS website. Renal clearance of inulin is the gold standard method for the determination of GFR; however, it is difficult to measure inulin in plasma and urine. On the other hand, it is relatively easy and therefore more practical to determine the renal clearance of creatinine (CrCl) as an approximation of GFR. CrCl is the volume of plasma cleared of creatinine per minute and is calculated by multiplying the concentration of creatinine in urine by the rate of urine production and then dividing the product by the serum creatinine concentration: For example, if the urine creatinine concentration is 60 mg/dL, urine production is 3 mL/min, and the serum creatinine concentration is 1.8 mg/ dL, then 100 mL of plasma is cleared of creatinine per minute. This value is divided by the animal’s body weight in kilograms and expressed in milliliters per minute per kilogram. Note that prerenal and postrenal factors, and also renal parenchymal lesions, will influence GFR. The major disadvantage of renal clearance studies is the requirement for timed urine collections. From a clinical standpoint, plasma clearance studies are less invasive and less time consuming. For example, plasma clearance of iohexol, an iodinated radiographic contrast agent, has been shown to estimate GFR reliably in dogs and cats. Iohexol plasma clearance is ideally performed in patients that are well hydrated and fasted for 12 h prior to the study. Iohexol is administered IV at a dosage of 300 mg iodine/kg body weight and then blood samples are collected at 2, 3, and 4 h after the IV injection from a separate vein. Serum from each blood sample is harvested (~1.5 mL of serum is needed per sample) and then transported either chilled or frozen to the specialized reference laboratory (e.g., Diagnostic Center for Population and Animal Health, Toxicology Section, Michigan State University) for measurement and calculation of GFR. Renal scintigraphy using 99mTc‐labeled diethylenetriaminepentaacetic acid also allows the GFR to be measured and is available at several universities in the United States and at major referral centers. This is a quick, non‐invasive method that does not require urine collection and has the advantage of being able to quantitatively evaluate individual kidney function. Disadvantages of this procedure include its limited availability, exposure of the animal to radioisotopes, the need for radioisotope disposal, and poorer correlation with inulin clearance compared with plasma iohexol clearance. Measuring the volume of urine produced is most frequently used as an aid in determining the maintenance fluid requirements of dogs and cats with AKI. Since these patients can range from markedly polyuric to completely anuric, actual quantification rather than estimation of output increases precision of assessment and fluid therapy. One major goal of fluid therapy in patients with AKI is to restore and/or maintain euvolemia to assure appropriate tissue (particularly renal) perfusion, while avoiding volume overload and its clinical consequences. Fluid therapy should be tailored to match urine volume plus other losses, including insensible losses (e.g., water loss due to respiration) and continuing losses (e.g., fluid loss due to vomiting or diarrhea). Insensible losses are estimated at 20 mL/kg/day. Urine output is quantitated at 6–8‐h intervals (best accomplished with indwelling or intermittent urinary catheterization) and that volume plus insensible loss is replaced over an equivalent subsequent time period. The volume of fluid loss due to vomiting and/or diarrhea may be estimated, and that amount is added to the 24‐h fluid needs of the patient. Fluid losses or gains can also be indirectly estimated by weighing the patient two to three times per day on the same scale. Biopsy and histopathologic evaluation of renal tissue are valuable diagnostic and prognostic tools, most often performed in cases of significant renal proteinuria or AKI. Though most management strategies for renal disease are broadly applicable to animals with nephropathies based on laboratory and physiologic parameters combined with measurable responses to therapy, applicability of certain targeted treatments depends on direct tissue examination. In addition, the prognosis for animals with renal disease will be most accurate and clinically useful when severity of dysfunction, renal histopathologic findings, and response to treatment can be examined together. Renal biopsy should be considered only after less invasive tests, often including response to therapies, have been carried out and coagulation/hemostasis has been assessed. Acquisition and examination of renal tissue is appropriate if the diagnosis is in question (e.g., glomerulonephritis versus amyloidosis), if treatment may be altered based on results (e.g., confirmation of and culture in bacterial pyelonephritis, immune‐mediated versus non‐immune‐mediated glomerulonephritis), or if the prognosis or client inclination to pursue further treatment may vary depending on findings (e.g., evidence of reversible tubular lesions in a dog or cat with AKI or presence of extensive fibrosis). Contraindications to renal biopsy include a solitary kidney, coagulopathy, severe systemic hypertension, late stage 3 and stage 4 CKD, and renal lesions associated with fluid accumulation (e.g., hydronephrosis, renal cysts, and abscesses). Very small patients (< 5 kg) may present additional challenge and risk for percutaneous biopsy procedures due to the smaller biopsy target, proximity of large vessels, and greater clinical impact of a given volume of hemorrhage [43]. Renal biopsy should not be attempted by inexperienced clinicians or in animals that are not adequately restrained. Specimens can be obtained percutaneously using the keyhole technique, or under laparoscopic or ultrasonographic guidance. Frequently, the best way to obtain renal tissue is at laparotomy when both kidneys can be visualized, postbiopsy hemorrhage can be accurately assessed and treated, and an adequate biopsy specimen assured. The cortical region of the kidney should be biopsied to obtain an adequate number of glomeruli in the specimen and to avoid renal nerves and major vessels at the corticomedullary junction and in the medullary region. Most animals will have microscopic hematuria for 1–3 days after the biopsy procedure, and transient gross hematuria is not uncommon. Severe hemorrhage is rare and is almost always the result of faulty technique. The International Veterinary Renal Pathology Service (IVRPS) housed at Texas A&M University is currently considered the nephropathologic standard for veterinary renal biopsy analysis. The IVRPS provides comprehensive sample analysis (light microscopy, transmission electron microscopy, and immunofluorescence evaluation), as well as histopathologic and clinical interpretation of results and their implications by nephropathologists and nephrologists. Information on ordering biopsy specimen collection kits, determining adequacy of samples acquired, and proper sample preparation and submission, as well as detailed information on when and how to appropriately biopsy a kidney is available online. Though they chiefly handle canine and feline samples, renal biopsies from other species are accepted. Even if a different pathology service is used, use of immunofluorescence and transmission electron microscopic assessment in addition to light microscopy is strongly recommended when evaluating renal tissue. Communication with the laboratory pathologist prior to biopsy will help determine which analytic modalities are available and which fixatives should be used to maximize utility of the biopsy sample. Prior to laboratory testing, physical exam findings can indicate renal dysfunction in horses. Common findings in horses with CKD include weight loss, polyuria/polydipsia, and ventral edema [8]. As in small animal patients, serum creatinine is a commonly used index to assess renal function (GFR). Similar to small animals, it is expected that serum creatinine elevations associated with renal lesions will not be observed until approximately 60–75% of the nephrons become non‐functional, and measurement of serum creatinine in early renal disease is of little value [44]. Breed may play a role in normal serum creatinine concentrations as heavily muscled breeds such as quarter horses have a greater upper limit in their normal reference range [44]. However, individual horses have minimal variation in serum creatinine concentrations making historical values particularly valuable for assessing change over time [45]. BUN measurement as a single test of renal function in horses has minimal diagnostic value. In horses, BUN is reabsorbed after filtration at the collecting ducts in a rate‐dependent manner based on the rate of fluid movement. As fluid rates and volumes increase in the collecting duct, less urea is reabsorbed; as fluid rates decrease, more urea is reabsorbed independent of the filtration rate of urea. Therefore, fluid therapy in horses with renal disease may reduce BUN but not in a manner that reflects improvement in GFR. Because serum creatinine is not reabsorbed, it is the most commonly used single test of renal function in horses. Azotemia, elevated serum creatinine and BUN, can be further divided into prerenal, renal, and postrenal in origin. The use of a BUN to serum creatinine (BUN:creatinine) ratio has been described for use in characterizing azotemia in horses and to separate acute and chronic forms of renal failure. The ratio is theoretically higher for prerenal azotemia because of increased urea reabsorption due to dehydration and low tubular flow rates compared with azotemia secondary to intrinsic renal disease. In horses with acute renal failure, BUN:creatinine ratios are less than 10:1, whereas in chronic disease, ratios can be expected to be greater than 10:1 [46]. GFR can also be measured in equine patients, providing a quantitative assessment of renal function; however, measurement of urine output is challenging in horses as an indwelling urinary catheter for urine collection is impractical. Serum electrolyte concentration measurements should also be considered in the diagnostic plan for horses with renal disease. Na+ and Cl– levels are often decreased in horses with renal disease, whereas K+ can be elevated, decreased, or normal. Hyperkalemia is most often observed with disease states associated with urine outflow obstructions or urinary bladder ruptures and subsequent uroperitoneum. Serum Ca2+ should also be monitored as horses with AKI are often hypocalcemic whereas those with CKD are often hypercalcemic. Urine samples should be obtained from horses with suspected renal disease for a complete urinalysis, including sediment examination. As in small animal patients, specific gravity should be measured along with microscopic examination for cells, casts, and crystals. The brush borders of the proximal tubular cells are rich in enzymes that play a role in the metabolic activity of the endothelium. Measured urinary GGT has been used, as a ratio with urine creatinine to determine and monitor the extent of acute tubular damage. GGT to urine creatinine ratios greater than 100 IU/g are considered to be clinically important, but lower ratios should be interpreted with caution as GGT release from the proximal tubules can occur with minor insults that may be clinically irrelevant. Fractional clearance of electrolytes may hold better diagnostic utility than urinary GGT for acute tubular damage [46]. The nephrons, as a collective group, work to conserve more than 99% of filtered Na+ and Cl− ions in the normal horse (supplemented salts or intravenous fluid administration will artificially increase the amount of excreted electrolytes in urine and, thus, complicate the utility of fractional clearance calculations). Increases in fractional clearance may be an indicator of early tubular damage. Serum and urine samples are collected simultaneously, and Na+, Cl−, and creatinine levels are determined. Using the following equation, a clearance value can be determined for sodium and chloride: where ClA/ClCr is the fractional clearance ratio, [A] is the measured electrolyte A (either Na+ or Cl−), and [Cr] is creatinine concentration. Symmetric dimethylarginine assays have been validated for use in horses, and reference ranges have been reported for adult horses and neonatal foals [47–49]. However, clinical utility in horses continues to be investigated and, to date, SDMA has not gained widespread use in equine patients. Renal biopsy via ultrasound guidance is easily performed in horses, and use of a needle biopsy instrument minimizes the risks (e.g., hemorrhage, bowel penetration, and peritonitis). Biopsy and histopathology can identify the type of lesion, location, and severity and provide guidance for prognosis. Many of the same diagnostic procedures performed in small animals and horses can be performed in ruminant species. However, for economic concerns, renal function testing is often limited to findings on routine chemistry analysis and urinalysis strips. The two major causes of AKI are toxic and ischemic injury, and the kidneys are highly susceptible to both due to their unique anatomic and physiologic features. For example, the large RBF (approximately 20% of the cardiac output) results in the increased delivery of bloodborne toxicants to the kidney compared to other organs. Within the renal cortex, the epithelial cells of the proximal tubule and thick ascending loop of Henle are most frequently affected by ischemic and toxicant‐induced injury. These particular susceptibilities occur, respectively, due to the many transport functions and consequent high metabolic rates of these cells, and to increased exposure from the combination of disproportionately high blood flow (the cortex receives 90% of the blood supplied by the renal artery) and the large endothelial surface area created by the glomerular capillaries. Toxicants disrupt the metabolic pathways that generate ATP, and ischemia can rapidly deplete cellular ATP stores. With the resulting loss of energy, the Na+‐K+ pump fails, leading to cell swelling and death. By resorbing water and electrolytes from the glomerular filtrate, tubular epithelial cells may be exposed to increasingly higher concentrations of toxicants within the tubular lumen. Toxicants that are either secreted or resorbed by tubular epithelial cells (e.g., gentamicin) may accumulate in high concentrations within these cells; similarly, the countercurrent multiplier system may concentrate toxicants in the medulla. Finally, the kidneys also play a role in the biotransformation of many drugs and toxicants. This usually results in the formation of metabolites that are less toxic than the parent compound; however, in some cases (e.g., the oxidation of ethylene glycol to glycolate and oxalate), the metabolites are more toxic than the parent compound. Toxic insults to the kidney are often caused by therapeutic agents, in addition to nephrotoxicants such as ethylene glycol and grapes/raisins. Similarly, ischemic insults to the kidney can occur in the hospital setting in conjunction with anesthesia and surgery or with the use of vasoactive agents or non‐steroidal anti‐inflammatory drugs (NSAIDs) [50]. Prolonged anesthesia with inadequate fluid therapy in older animals with pre‐existing, subclinical CKD is a frequent cause of renal ischemia and AKI in the hospital setting. Normal kidneys can maintain adequate renal perfusion pressure by autoregulation provided that the mean arterial blood pressure exceeds approximately 60–70 mmHg. This autoregulation may be compromised, however, in patients with pre‐existing CKD, especially during anesthesia. The resulting decline in RBF and perfusion pressure can adversely affect GFR and delivery of oxygen and nutrients to the metabolically active tubular epithelial cells. Tubular cell swelling secondary to decreased Na+‐K+ pump activity occurs due to osmotic extraction of water from the extracellular space, which, in turn, can cause the amount of water in the plasma to decrease. The consequences of decreased plasma water in the renal vasculature are red blood cell aggregation and vascular congestion and stasis, which tend to potentiate and perpetuate decreased glomerular blood flow and decreased oxygen and nutrient delivery.
43
Physiology, Pathophysiology, and Anesthetic Management of Patients with Renal Disease
Introduction
Normal anatomy and physiology
Anatomy of the urinary tract
Physiologic function
Nephron structure
Major activity
Filtrate composition
Comments
Glomerulus
Filtration of plasma
Isotonic
Cell‐free filtrate containing only fluid, electrolytes, and small proteins
Proximal convoluted tubule
45–55% of filtrate is reabsorbed
Isotonic
90% of HCO3− is reabsorbed and filtrate becomes acidic
Descending loop of Henle
25–40% of filtrate H2O is reabsorbed
Hypertonic
H2O reabsorption via osmosis
Ascending loop of Henle
Major area of solute reabsorption
Hypotonic
Active transport via Na+‐K+ ATPase, cells are impermeable to H2O
Distal convoluted tubule
5% of filtrate is reabsorbed
Hypotonic
Less than 10% of originally filtered H2O is present
Collecting duct
0.5–10% of H2O reabsorption
Hyper‐, iso‐, or hypotonic (urine)
Under the influence of arginine vasopressin
Testing and monitoring renal function
Canine and feline
Biochemical testing
Renal clearance and glomerular filtration rate
Urine output measurement
Renal biopsy
Equine
Ruminant
Acute kidney injury/acute renal failure
Etiology and pathogenesis
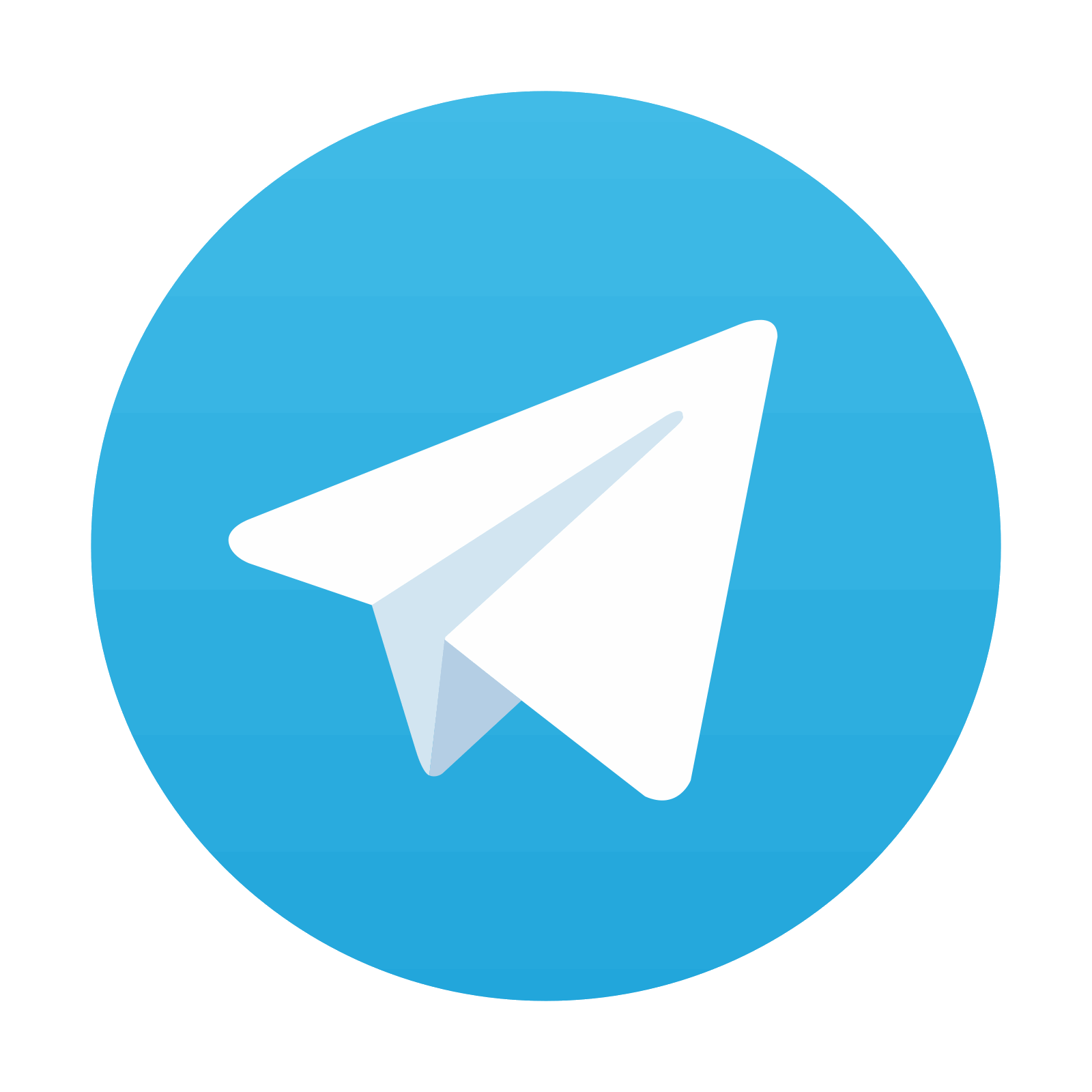
Stay updated, free articles. Join our Telegram channel
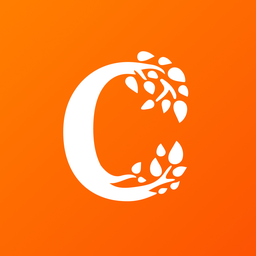
Full access? Get Clinical Tree
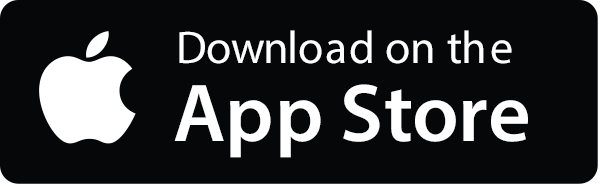
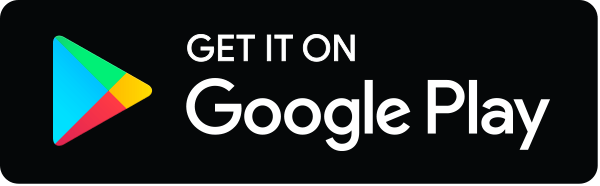