39Physiology, Pathophysiology, and Anesthetic Management of Patients with Neurologic Disease
Tatiana H. Ferreira1 and Starr Cameron2
1 Department of Surgical Sciences, School of Veterinary Medicine, University of Wisconsin, Madison, Wisconsin, USA
2 Department of Medical Sciences, School of Veterinary Medicine, University of Wisconsin, Madison, Wisconsin, USA
Introduction
Anesthetic management of patients with neurological disorders undergoing diagnostic and/or surgical procedures is common in veterinary medicine. Knowledge of normal anatomy and physiology as well as pathophysiology of the central, peripheral, somatic, and autonomic nervous systems allows the anesthetic provider to understand the impact of sedation and anesthesia on normal physiology as well as disease processes. Whether a patient is anesthetized for evaluation or management of neurologic conditions or suffers from comorbidities related to the nervous system, a solid foundational knowledge of the nervous system is important to ensure appropriate and optimal patient care while decreasing patient morbidity and mortality.
Anatomy of the nervous system
The nervous system is anatomically divided into the central nervous system, containing the brain and spinal cord, and the peripheral nervous system, containing the cranial and spinal nerves. Functionally, the nervous system can be divided into somatic and autonomic nervous system components, each with afferent and efferent fibers.
Central nervous system
The central nervous system (CNS) contains the brain and spinal cord and is made up of gray and white matter. Gray matter is composed of neuronal cell bodies and is located more superficially in the brain and more deeply (i.e., centrally) within the spinal cord. There are also some deeper regions of gray matter within the brain known as “nuclei.” White matter is composed of nerve fibers (axons) and is located deeper within the brain and more superficially within the spinal cord. The meninges are composed of three layers of connective tissue within the CNS: the dura, arachnoid, and pia mater. The dura mater is the most superficial layer and, in the vertebral column, the space between the bone and the dura mater is the epidural space. Within the skull, the dura mater is fused to the periosteum and, therefore, is considered a potential space. Because there is no epidural space intracranially, the venous sinuses are located within the dura mater of the brain. The arachnoid is the middle layer of the meninges while the pia mater is the innermost layer. The pia mater is only one cell thick and is fused to the surface of the brain and the spinal cord. Within the vertebral canal, the subarachnoid space is located between the arachnoid and pia mater layers and contains cerebrospinal fluid (CSF). The meninges extend around the spinal nerve roots with some reaching the level of the intervertebral foramina.
When CSF collection is performed, a spinal needle is inserted into the subarachnoid space either at the level of the cerebellomedullary cistern cranially, or the lower lumbar region caudally. In dogs and cats, the L5–L6 vertebral space is recommended for obtaining CSF. In large‐breed dogs, the L4–L5 vertebral space can often be used if obtaining CSF from the L5–L6 space is unsuccessful. Local anesthetic and analgesic agents may be administered either in the epidural or subarachnoid spaces; species‐specific descriptions of these techniques may be found in the local anesthetic and analgesic techniques chapters in this text.
Brain
Anatomically, the brain can be divided into five regions: the telencephalon (cerebral cortex and basal ganglia), diencephalon (thalamus, hypothalamus, epithalamus, subthalamus, and metathalamus), mesencephalon (midbrain), metencephalon (pons and cerebellum), and myelencephalon (medulla oblongata) (Fig. 39.1). Functionally and clinically, the telencephalon and diencephalon together make up the prosencephalon or forebrain. The midbrain, pons, and medulla form the brainstem. There are 12 pairs of cranial nerves; a list of the nerves and their innervation is provided in Table 39.1.
The cerebral cortex or telencephalon is where sensory information from the environment is consciously perceived and voluntary motor functions are initiated. The telencephalon is divided into right and left hemispheres. In mammals, except mice and rats, the cerebral cortex is composed of gyri and sulci and classified as gyrencephalic. The brains of fish, reptiles, birds, mice, and rats are smooth and lack these characteristic folds and are referred to as lissencephalic. The presence of gyri allows a significantly increased number of neurons without increasing the size of the cranium or skull. The gyri are divided into lobes including the frontal, parietal, temporal, and occipital lobes. The olfactory bulb is the most rostral part of the telencephalon and is where the axons of the olfactory nerves terminate.

Figure 39.1 Sagittal section of the canine brain. Teg, tegmentum.
Source: Jenkins [1], with permission.
Table 39.1 Cranial nerves and their innervation.
Cranial nerve number | Cranial nerve name | Classification | Function/innervation |
---|---|---|---|
I | Olfactory | Sensory | Olfaction |
II | Optic | Sensory | Vision |
III | Oculomotor | Motor | Somatic motor information to dorsal, medial, ventral rectus, ventral oblique, and levator palpebrae superioris muscles of the eyeParasympathetic innervation to pupil (constriction) |
IV | Trochlear | Motor | Somatic motor innervation to dorsal oblique muscle of eye |
V | Trigeminal | Mixed | Somatic motor innervation to muscles of mastication and tensor tympani muscleSensory innervation to most of the face and muzzle |
VI | Abducens | Motor | Somatic motor innervation to lateral rectus and retractor bulbi muscles of the eye |
VII | Facial | Mixed | Somatic motor innervation to muscles of facial expression and stapedius musclesSensory innervation of inner pinnaeSensory innervation to rostral two‐thirds of tongueParasympathetic innervation to salivary, lacrimal, palatine, and nasal glands |
VIII | Vestibulocochlear | Sensory | Vestibular function and hearing |
IX | Glossopharyngeal | Mixed | Somatic motor innervation to larynx and pharynxSensory innervation of pharynx and caudal third of tongueParasympathetic innervation of salivary glands (parotid and zygomatic) |
X | Vagus | Mixed | Somatic motor innervation to larynx and pharynxSensory innervation of pharynxParasympathetic innervation to viscera |
XI | Accessory | Motor | Somatic motor innervation to larynx |
XII | Hypoglossal | Motor | Somatic motor innervation to the tongue |
The diencephalon, specifically the thalamus, receives all sensory information (except olfaction) and projects to sensory areas of the telencephalon. Efferent, or motor, pathways also course from the cerebral cortex through the thalamus to the brainstem. The hypothalamus is important in maintaining homeostasis as it is the neurologic control center for the endocrine system and the autonomic nervous system. The axons of cranial nerve II (optic nerve) terminate within the diencephalon after coursing through the optic chiasm.
The mesencephalon, or midbrain, is the most rostral part of the brainstem. The midbrain contains sensory and motor tracts, as well as the rostral and caudal colliculi, which are associated with visual and auditory reflexes, respectively. The nuclei of cranial nerves III (oculomotor nerve) and IV (trochlear nerve) are located within the mesencephalon. The midbrain is connected to the cerebellum via the rostral cerebellar peduncles.
The metencephalon, or pons, also contains sensory and motor pathways and the nuclei associated with cranial nerve V (trigeminal nerve). The cerebellum is also part of the metencephalon and is located dorsal to the brainstem. The pons is connected to the cerebellum via the middle cerebellar peduncles. The cerebellum has two hemispheres and a centrally located vermis. It receives sensory information directly from afferent pathways, including the vestibular system, and motor information from the cerebral cortex. While the cerebellum does not initiate movement, it is responsible for integrating sensory and motor input to create smooth, controlled, and coordinated movements.
The myelencephalon, or medulla, contains sensory and motor tracts, as well as nuclei for the remaining pairs of cranial nerves VI through XII, including cranial nerve X (vagus nerve), which innervates organs within the thorax and abdomen. In addition, nerve centers for vital body functions, including regulation of blood pressure, heart rate, and respiration, are located within the medulla.
The blood–brain barrier (BBB) is a semipermeable membrane made up of microvascular endothelial cells that restrict free movement of particles from the blood into the brain parenchyma and CSF. The BBB allows carbon dioxide, oxygen, small lipid‐soluble substances, and general anesthetics to cross from the blood into the brain. The BBB is made up of pericytes, tight junctions between capillary endothelial cells, a layer of astrocyte foot processes that surround the capillaries, and the basal lamina (or basement membrane). Several areas of the brain, including the hypothalamus, area postrema, and the pineal gland, are outside the BBB as these regions contain sensors for monitoring osmolarity, glucose levels, and pH, and are important for providing information for maintenance of homeostasis. While the BBB fulfills a protective function by preventing possible toxins and limiting pathogens from entering the CNS, it also limits therapeutic options by excluding many drugs from entering. Permeability of the BBB may increase in the face of inflammation or increased intracranial pressure.
The CSF is a clear, colorless fluid produced by the choroid plexus and the ependymal lining cells within the ventricles of the brain and is an ultrafiltrate of plasma. It is produced at a rate of 0.02 mL/min in cats and 0.05 mL/min in dogs [2,3]. Compared to plasma, CSF has a lower concentration of protein, glucose, calcium, and potassium and a higher concentration of sodium, chloride, and magnesium [4]. The CSF is present within the lateral, third, and fourth ventricles of the brain, and within the central canal and subarachnoid space of the spinal cord. The CSF flows from the lateral ventricles, located within the cerebral cortex, into the third ventricle, located between the thalamus and hypothalamus. From here, it flows into the fourth ventricle, located ventral to the cerebellum and dorsal to the medulla. These ventricles can be clearly seen on magnetic resonance imaging (MRI) (Fig. 39.2). Caudally, the fourth ventricle becomes continuous with the central canal of the spinal cord and bilateral apertures within the ventricle allow CSF to flow into the subarachnoid space. The CSF passes from the subarachnoid space through arachnoid villi, which act as one‐way valves, to the dural sinuses where it is absorbed. The arachnoid villi are located within the venous sinuses, as well as the spinal veins.
The blood supply to the arterial circle, or the “Circle of Willis” as termed in human medicine, is the main arterial supply to the brain. There are several anatomical species differences that can have clinical implications. In dogs (and in humans), the arterial circle is formed by the joining of the basilar artery and the left and right internal carotid arteries. In the cow, the maxillary and vertebral arteries form this arterial supply. In the cat and sheep, the maxillary artery, via the rete mirabile, forms the arterial circle. The rete mirabile is a network of branches of the maxillary artery located in the cavernous sinus on the ventral surface of the calvarium. The blood from the cavernous sinus drains from the nasal cavity. A countercurrent heat exchange mechanism between the rete mirabile (arterial) and cavernous sinus (venous) may play a role in thermoregulation of the brain. Because of this anatomic variation, using a spring‐held mouth gag in cats may increase the risk of neurologic complications associated with anesthesia, including cortical blindness and hearing deficits [5–7]. One study identified changes in electroretinogram (ERG) and brainstem auditory evoked responses (BAER) in anesthetized cats when mouth gags were used compared to no changes when mouth gags were not used [8]. From the arterial circle, the rostral, middle, and caudal cerebral arteries supply the prosencephalon. The blood supply to the brainstem arises as branches from the basilar artery.

Figure 39.2 T2‐weighted magnetic resonance imaging (MRI) sagittal views. A. Normal canine brain. B. Feline brain with signs of foramen magnum (arrow) and transtentorial (arrowhead) herniation secondary to a large intracranial tumor.
Source: Images courtesy of Dr. Starr Cameron.
The venous system including the venous sinuses and veins of the CNS is valveless, meaning blood can flow in either direction. Associated vessels have thin walls without muscular layers and the veins drain into the larger venous sinuses. The cerebral cortex contains the dorsal and ventral cerebral veins as well as the great cerebral vein which drains the deeper cortex. The major veins of the brainstem include the dorsal and ventral cerebellar veins, pontine veins, and medullary veins. The dorsal sagittal sinus, straight sinus, and paired transverse sinuses are located dorsally. Paired rostral and caudal cavernous sinuses, as well as the sigmoid, basilar, and petrosal sinuses, are located ventrally within the calvarium. The venous sinuses are located within the meninges and drain into the internal jugular, maxillary, and vertebral veins and into the venous plexus.
Spinal cord
Rostrally, the spinal cord is continuous with the myelencephalon, or medulla, of the brainstem and is a complex network of ascending and descending tracts. It is located within the spinal, or vertebral, canal and is surrounded by the meninges, which are composed of the dura mater, arachnoid, and pia mater. The epidural space contains epidural fat, blood vessels, and lymphatics. The CSF passes through the central canal of the spinal cord and flows around the spinal cord within the subarachnoid space. The pia mater is one cell thick and lies directly on the spinal cord.
Anatomically, the spinal cord is divided longitudinally into four major regions: cervical, thoracic, lumbar, and sacral. The caudal (coccygeal) segments are associated with the tail and contain nerves. Each segment of the spinal cord has a pair (right and left) of spinal nerves that exit through the intervertebral foramen. The dorsal root carries sensory (afferent) information and the ventral root carries motor (efferent) information. Just inside the intervertebral foramina, the dorsal and ventral roots combine and exit as the associated spinal nerve for that segment of spinal cord. The number of vertebrae and spinal cord segments varies with species. Spinal nerve C1 exits through a foramen in C1 and the spinal nerves C2–C7 exit cranial to the associated vertebrae. Spinal nerve C8 exits between C7 and T1, and spinal nerves T1–L3 exit caudal to the associated vertebrae. The vertebrae and spinal cord segments are misaligned to varying degrees due to differential growth rates during development. For example, in the lumbar region of the dog, spinal cord segments L5, L6, and L7 all lie within the region of the L4 vertebra. In medium to large‐breed dogs, the spinal cord terminates at approximately L6–L7, and in small‐breed dogs and cats, the spinal cord terminates at approximately S1. In large animals, including horses, ruminants, and swine, the spinal cord terminates mid‐sacrum. These anatomic differences are important to note when considering diagnostic and therapeutic procedures involving the subarachnoid and epidural spaces.
Clinically and functionally, the spinal cord is divided into four segments based on innervation: C1–C5, C6–T2, T3–L3, and L4–S3. The cervical (C6–T2 segments) and lumbosacral (L4–S3 segments) intumescences supply the lower motor neurons to the thoracic and pelvic limbs, respectively. Lesions within the cervical segments (C1–C5 and C6–T2) can cause tetraparesis, hemiparesis, tetraplegia, or hemiplegia. Cervical pain is also common with lesions in this region but may or may not be present. Lesions within the T3–L3 or L4–S3 segments may cause paraparesis or paraplegia and, depending on the etiology, lesions may cause back pain (Fig. 39.3).
Caudal to the lumbar intumescence, the spinal cord tapers into a region called the “conus medullaris,” which consists of sacral and caudal segments. The filum terminale is a strand of glial and ependymal cells that extends from the end of the spinal cord. This region is covered by a dural sac, creating a space within the subarachnoid space filled with CSF. As the sacral and caudal spinal roots move caudally past the conus medullaris, each exits at an intervertebral foramen. These roots are collectively known as the “cauda equina.”
The spinal cord has an H‐ or butterfly‐shaped center of gray matter, which is divided into the dorsal, lateral, and ventral horns (Fig. 39.4). The dorsal horn contains sensory neurons. The lateral horn is located in the thoracolumbar (T1–L4, specifically) and sacral segments and contains motor neurons that innervate the viscera. The ventral horn contains cell bodies of somatic motor neurons, which are also called lower motor neurons. The white matter surrounds the gray matter of the spinal cord and is divided into three funiculi. The dorsal funiculus contains only sensory fiber tracts in several mammalian species including the dog, while the lateral and ventral funiculi carry both sensory and motor fiber tracts.
Cranially, the subclavian artery branches to become the vertebral artery, which enters the vertebral canal at the atlas (first cervical vertebra). Segmental spinal arteries enter the spinal cord via the intervertebral foramen where they divide into dorsal and ventral branches. The ventral branches connect via the longitudinal ventral spinal artery (which runs in the ventral median fissure of the spinal cord) and supply the ventral gray matter and ventral funiculus. The dorsal branch supplies the dorsal gray matter. Other branches of the segmental spinal arteries supply the lateral funiculus. Blood flow in the gray matter of the spinal cord is four times that of the white matter, and blood flow to the thoracolumbar region of the spinal cord has been shown to be 40% less compared to the cervical and lumbosacral regions [9]. There is less gray matter in the thoracolumbar region of the spinal cord, which accounts for the reduced blood flow.
The venous system of the CNS is valveless and vertebral veins communicate directly with the cranial venous sinus described above. Lying ventral to the spinal cord is the internal vertebral venous plexus, which extends the entire length of the spinal canal. This flat, thin‐walled valveless network is paired and is a frequent source of hemorrhage during neurosurgery. The sinus diverges at the intervertebral foramen and then converges over the vertebral body. At the level of the intervertebral foramen, the sinus communicates with the intervertebral veins and exits the foramen with the spinal nerve roots where they drain into larger vessels, including the azygous vein and caudal vena cava.
Peripheral nervous system
The peripheral nervous system (PNS) is divided into afferent (sensory) and efferent (motor) components. Sensory neurons receive information from peripheral receptors and transmit this information via action potentials to the CNS for integration and interpretation. Sensory neurons enter the intervertebral foramen as part of the spinal nerve and then enter the dorsal horn of the spinal cord via the dorsal nerve root. Efferent information leaves the ventral horn of the spinal cord via the ventral nerve root before becoming the spinal nerve and then motor neurons. There are two divisions of motor neurons within the PNS: somatic motor neurons, which innervate skeletal muscle, and autonomic motor neurons, which innervate cardiac muscle, smooth muscle, and some glands.

Figure 39.3 T2‐weighted magnetic resonance image (MRI) sagittal views. A. Cervical spine in a cat with an intramedullary C1–C2 myelopathy (arrow) most consistent with feline ischemic myelopathy. B. Thoracic spine in a dog with an acute intervertebral disc extrusion at the T13–L1 disc space (arrow).
Source: Images courtesy of Dr. Starr Cameron.

Figure 39.4 Transverse section of the spinal cord within the vertebral canal. Note the adipose tissue and blood vessels in the epidural space.
Source: Jenkins [1], with permission.
Myelin, produced by oligodendrocytes within the CNS and by Schwann cells within the PNS, insulates the axons of nerves and expedites transmission of electrical activity. The nodes of Ranvier, which are the spaces or gaps between myelin along the axon, allow saltatory conduction to take place which greatly expedites signal transmission. Therefore, nerves that are myelinated have considerably faster conduction velocities compared to unmyelinated nerves. In addition, large fiber nerves also have faster conduction velocities compared to small fiber nerves. The largest and fastest nerve fibers are called Type A and innervate somatic nerves. Type B fibers are also myelinated but are smaller than Type A and make up the preganglionic nerves of the autonomic nervous system. Type C are small unmyelinated nerve fibers with the slowest conduction velocity. Type C fibers transmit postganglionic information within the autonomic nervous system, as well as sensory information regarding temperature, pain, and touch.
Somatic nervous system
The somatic nervous system contains both afferent (sensory) and efferent (motor) nerves. Afferent fibers transmit environmental and peripheral sensory information regarding pain, temperature, and pressure, as well as sight and hearing. The cutaneous region innervated by one specific sensory spinal nerve is called a dermatome, and the presence or absence of sensation in dermatomes can be useful to isolate a particular sensory nerve that is affected by a lesion. Skeletal muscles contain specialized sensory receptors. Muscle spindles provide information on muscle length and are located within the extrafusal fibers of skeletal muscles. Golgi tendon organs provide information about muscle tension and are located within the tendons. All of these are examples of the information transmitted by the general somatic afferent system.
Neuromuscular junction transmission
Motor neurons are found in both cranial and spinal nerves and form synapses with skeletal, smooth, or cardiac muscle fibers at the neuromuscular junction. The motor endplate is the specific region where the nerve meets the muscle, with most muscle fibers having one neuromuscular junction per muscle fiber. The neurotransmitter acetylcholine (ACh) is released from the presynaptic neuron and crosses the synaptic cleft to bind to receptors on the postsynaptic muscle membrane. Depending on the strength of the stimulus and the amount of ACh released, this action potential may cause muscle contraction. Even without action potentials, ACh is constantly being released in small amounts which causes miniature endplate potentials.
In a resting state, there is an excess of sodium ions outside the muscle membrane and an excess of potassium ions inside the muscle membrane, creating a negatively charged environment inside the muscle. The electrical difference between the two sides of the membrane is 90 mV. Once a nerve is stimulated, an action potential travels down the axon and reaches the presynaptic terminal of the nerve where ACh is present in small synaptic vesicles. These vesicles fuse with the presynaptic membrane and ACh is released. ACh diffuses across the cleft and reaches the postsynaptic muscle membrane where it binds reversibly to nicotinic receptors. There are five subunits of the nicotinic receptor, and when two ACh molecules bind to the two α subunits, an allosteric change in conformation of the membrane proteins occurs. Sodium ions flow into the muscle membrane and potassium ions flow out of the muscle membrane, causing a change in the electrical charge, which is called depolarization. If the change in charge inside the muscle membrane is greater than 45 mV, then muscle contraction usually occurs. There is an overabundance of ACh released by an action potential into the neuromuscular junction, which acts as a safety margin to ensure adequate binding. Excess ACh is broken down by the enzyme acetylcholinesterase into acetic acid and choline and then recycled and returned to the presynaptic nerve. The impulses are transmitted through the T‐tubules of the sarcolemma of the muscle membrane, causing a release of calcium from the sarcoplasmic reticulum and muscle contraction.
In addition to the presynaptic nerve and postsynaptic muscle receptors described above, there are other postsynaptic receptors located outside the neuromuscular junction on the muscle membrane and these are called extrajunctional (or extrasynaptic) receptors. These receptors, which normally play a very small role in neuromuscular transmission, proliferate after denervation or muscle injury and may cause an exaggerated response that can be detected after a depolarizing neuromuscular blocking agent (i.e., succinylcholine) has been administered.
Autonomic nervous system
The autonomic nervous system (ANS) is responsible for regulating the body’s internal environment and helping to maintain homeostasis. Most of the functions of the ANS are under subconscious control and include maintaining heart rate and blood pressure, thermoregulation, controlling micturition, and managing gastrointestinal secretions, although the gastrointestinal tract does have additional nervous system components. The ANS consists of afferent pathways, CNS integration (brain and spinal cord), and two efferent pathways: the sympathetic (flight or fight) and parasympathetic (rest and digest) systems.
Within the diencephalon, the hypothalamus facilitates regulation of both the sympathetic and parasympathetic nervous systems, along with the cerebral cortex and brainstem. Both systems are always active, and activity can be increased or decreased to control organ function and response. Most organs have both sympathetic and parasympathetic innervation, creating reciprocal effects. For example, the heart has sympathetic innervation which increases heart rate and contractility, and parasympathetic innervation which has opposing effects. Sympathetic innervation of the gastrointestinal tract causes relaxation while parasympathetic innervation causes contraction. Unlike the somatic system which has one efferent neuron, efferent information from the ANS is conducted via two (preganglionic and postganglionic) neurons. The preganglionic neuron is within the CNS, and the postganglionic neuron is within the PNS.
The general visceral afferent fibers carry afferent (sensory) information to the CNS and that information is then used to control internal homeostasis and bodily functions via the efferent components of the ANS. Afferent information from cranial nerves VII, IX, and X travels along the solitary tract within the medulla and synapses within the solitary nucleus. Axons then travel to other regions of the brainstem (parasympathetic system) or spinal cord (sympathetic system) to control visceral functions or project to the thalamus for conscious perception of sensory information. Afferent information regarding taste travels via cranial nerves VII and IX. Blood pressure and tissue oxygenation levels are monitored by baroreceptors and chemoreceptors located in arteries and information is transmitted to cranial nerves IX and X. Additionally, sensory information from the olfactory and limbic regions is carried along the solitary tract to the solitary nucleus to allow the ANS to respond to smells and emotions, respectively.
ACh is the neurotransmitter for all preganglionic synapses within the sympathetic and parasympathetic systems, as well as for postganglionic synapse transmission in the parasympathetic system. In postganglionic sympathetic synapses, norepinephrine is the main neurotransmitter secreted; however, some sympathetic postganglionic synapses, such as those that supply sweat glands and blood vessels of skeletal muscles, secrete ACh. ACh receptors are either nicotinic or muscarinic. Nicotinic receptors are found in all preganglionic synapses of the ANS and at the somatic neuromuscular junction. Muscarinic receptors are located in effector cells at postganglionic synapses of the parasympathetic nervous system and in cholinergic postganglionic synapses of the sympathetic nervous system. A list of selected effects of sympathetic and parasympathetic nervous system transmission can be found in Table 39.2.
Table 39.2 Selected effects of sympathetic and parasympathetic nervous system transmission.
Organ | Sympathetic | Parasympathetic | ||
---|---|---|---|---|
Action | Receptor | Action | Receptor | |
Heart Rate Contractility | IncreaseIncrease | β1β1 | DecreaseDecrease | MM |
Blood vessels Arteries Veins Skeletal muscle | VasoconstrictionVasoconstrictionVasodilation | α1α2β2 | ||
Bronchioles | Bronchodilation | β2 | Bronchoconstriction | M |
Salivary glands | Increase secretion | α1 | Increase secretion | |
Gastrointestinal tract | Relaxation | α2 | Contraction | M |
Kidney | Renin secretion | β1 | ||
Ureter | Contraction | α1 | Relaxation | M |
Urinary bladder | Relaxation | β2 | Contraction | M |
Internal urethral sphincter | Contraction | α1 | Relaxation | M |
Uterus | Contraction | α1 | Variable | |
Eye Iris dilator muscle Iris sphincter muscle Ciliary muscle | Contraction (mydriasis)Relaxation | α1β2 | Contraction (miosis)Contraction | MM |
Adipose tissue | Lipolysis | β1 | ||
Sweat glands | Increase secretion | α1 | ||
Hair follicles | Contraction (piloerection) | α1 |
M, muscarinic.
Sympathetic nervous system
Often referred to as the “fight or flight system,” the sympathetic nervous system is most active during times of stress. It discharges as a unit, and the effects are widespread and last longer than those of the parasympathetic system. ACh is the neurotransmitter between preganglionic and postganglionic neurons within the sympathetic system. Norepinephrine is the main neurotransmitter used between postganglionic neurons and the effector organ within the sympathetic nervous system. Norepinephrine acts as an agonist at α1‐, α2‐, β1‐, and β2‐adrenergic receptors. For sweat glands, the receptor is cholinergic, except in horses where the receptor is β2‐adrenergic [10].
Visceral sensory (afferent) information travels along sympathetic nerves to the dorsal horn of the spinal cord. There, interneurons receive information from visceral and somatic afferent nerves. The sympathetic system has short preganglionic and long postganglionic axons. The efferent preganglionic neurons of the sympathetic nervous system are located within the gray matter of the thoracolumbar spinal cord, specifically the T1–L5 spinal cord segments (Fig. 39.5). The axons leave via the ventral nerve root to join the spinal nerve of the associated spinal cord segment and then synapse in a ganglion at one of three locations. Some preganglionic neurons synapse in the sympathetic chain ganglion located along the lateral aspect of the vertebral column (paravertebral). These synapses can occur within ganglia at the same segmental level of the chain, or they may synapse rostrally or caudally which allows for multiple possible synapses. This more diffuse distribution accounts for the widespread effects of sympathetic innervation. The postganglionic neurons then travel to innervate blood vessels, sweat glands, and pilomotor muscles. Other preganglionic neurons travel through the sympathetic chain without synapsing. These neurons then synapse on prevertebral ganglia that supply visceral organs and glands within the thoracic or abdominal cavities. The prevertebral ganglia include the celiac, cranial, and caudal mesenteric ganglia.
Lastly, other preganglionic neurons of the sympathetic nervous system travel from the thoracolumbar region of the spinal cord via the splanchnic nerve to the adrenal medulla where they synapse on chromaffin cells. These neurons release ACh which acts at nicotinic receptors located on chromaffin cell membranes, stimulating these cells to release epinephrine (80% in dogs, 60% in cats) and norepinephrine (20% in dogs, 40% in cats) into circulation [11]. Epinephrine causes vasodilation within skeletal muscles and bronchodilation within the airways, while norepinephrine causes vasoconstriction, increased cardiac activity, and pupillary dilation.
Parasympathetic nervous system
The parasympathetic nervous system of the ANS also has preganglionic and postganglionic neurons. In contrast to the sympathetic preganglionic fibers, the efferent parasympathetic preganglionic axons are long and tend to synapse either within or very near the target organ, meaning that the postganglionic fibers are quite short. The parasympathetic nervous system efferent nuclei are located within the brainstem or sacral spinal cord segments, leading this portion of the ANS to be referred to as the craniosacral component. The preganglionic nuclei of the parasympathetic nervous system are located within the nuclei of cranial nerves III, VII, IX, and X, adjacent to the somatic nuclei of these cranial nerves (Fig. 39.5). The preganglionic parasympathetic nucleus (often called the “Edinger–Westphal nucleus”) of the oculomotor nerve (cranial nerve III) is located within the midbrain. The postganglionic neuron stimulates the iris (pupillary) sphincter and ciliary muscles of the eye to cause pupillary constriction. Postganglionic fibers from the facial and glossopharyngeal nerves (cranial nerves VII and IX, respectively) innervate salivary glands. The parasympathetic nucleus of the vagus nerve (cranial nerve X) provides efferent control to the heart, lungs, gastrointestinal tract, liver, pancreas, and gallbladder.

Figure 39.5 The sympathetic and parasympathetic nervous systems. Note the distribution of cranial nerves III, VII, V, IX, and X.
The sacral segments of the parasympathetic nervous system regulate bladder control, the internal urethral sphincter, smooth rectal muscles, and the reproductive organs. During micturition, postganglionic parasympathetic neurons cause detrusor muscle contraction and urethral relaxation for urine voiding.
Preganglionic neurons of the parasympathetic system release ACh which acts on nicotinic receptors of the postganglionic neurons. Postganglionic neurons also release ACh but the receptors at the effector organs are mostly muscarinic.
Physiology of the central nervous system
Brain physiology
Cerebral perfusion pressure and intracranial pressure
According to the Monro–Kellie doctrine, the brain is enclosed in the rigid structure of the skull and an equilibrium must exist between the volumes of brain parenchyma, interstitial and CSF fluid, and arterial/venous blood; therefore, an increase in one component or the addition of another (e.g., hematoma, skull fracture, and tumor) must cause a decrease in the remaining components [13,14]. Additionally, an increase in the volume of any constituent can result in an increase in intracranial pressure (ICP). Blood and CSF are the main volume buffering components; thus, initial compensatory mechanisms such as a decrease in cerebral blood volume (CBV), and/or a shift of CSF from the brain into the spinal canal, or even absorption of CSF will prevent major increases in ICP [15]. This stage is often characterized as the compensated state of increased ICP. While changes in cerebral venous volume occur rapidly, “spatial compensation” of CSF happens more slowly, so its ability to mitigate acute increases in ICP is limited [14,16]. Therefore, once these compensatory mechanisms are exhausted, marked elevations in ICP can occur leading to decreased cerebral perfusion pressure (CPP), defined as the difference between mean arterial blood pressure (MAP) and ICP (i.e., CPP = MAP – ICP), and increased risk of cerebral ischemia [14,15]. Significant increases in ICP can also result in compression of brain tissue against the tentorium, falx, and foramen magnum and potential brain herniation, which is characterized as the decompensated state of increased ICP [15,16].
Some causes of increased ICP include space‐occupying lesions (i.e., intracranial hemorrhage, hematoma, tumor, abscess), cerebral edema (i.e., due to head injury, or hypoxic or ischemic encephalopathy), venodilation, and a decrease in venous drainage (i.e., head‐down position, jugular vein obstruction/compression, and increased right heart or intrathoracic pressures) [14–17]. In addition, increased CSF production (i.e., choroid plexus tumor) or impeded CSF reabsorption or outflow (i.e., flow obstruction by space‐occupying lesions and Chiari malformation) can result in an increase in CSF volume and ICP [14,15].
As a result of increased ICP, a physiologic response known as the “Cushing reflex” occurs, which has classically been reported as an increase in systolic blood pressure, bradycardia, and an irregular (Cheyne–Stokes) breathing pattern [18–21]; however, subsequent studies led to refinement of Cushing’s original findings [22]. Initially, both blood pressure and heart rate may rise due to sympathetic activation [22–24] as MAP increases to maintain CPP. Over time, hypertension persists in the face of elevated ICP, but heart rate begins to decrease. The mechanism resulting in bradycardia continues to be debated with some suggesting it is a baroreceptor reflex while others propose it is a result of intracranial compression of the vagus nerve [24]. Finally, once the brainstem is compressed and the respiratory center is compromised by increased ICP, irregular breathing and even apnea (respiratory arrest) can ensue [24]. The Cushing reflex has also been recognized in cases of decreased CPP (< 15 mmHg) in humans [25]. As bradycardia is a late component of the physiologic response, focusing on this symptom may result in the patient being subjected to a prolonged period of intracranial hypertension prior to intervention, resulting in a higher incidence of complications [25]. Therefore, in the human literature, monitoring for systemic hypertension and tachycardia as potential early indicators of increased ICP and impaired brain perfusion has been recommended to facilitate earlier intervention and improved outcomes [25].
Regulation of cerebral blood flow
The brain is an organ with high metabolic demand. Despite this high demand for energy substrates (mainly oxygen and glucose) to maintain homeostasis, extreme fluctuations in cerebral blood flow (CBF) are not tolerated due to the non‐compliant cranium and meninges. Thus, tight control and regulation of tissue pressure (i.e., ICP) is required because increases in CBV will lead to increases in ICP, and vice versa [26,27]. Several key factors contribute to regulation of CBF including the arterial partial pressure of carbon dioxide (PaCO2), MAP, cerebral metabolic rate (CMR), and the ANS [27]. For simplicity, regulators of CBF may be classified as chemical, myogenic, and neurogenic factors [26].
Chemical regulation of cerebral blood flow
Chemical regulation involves changes in CMR, PaCO2, and arterial partial pressure of oxygen (PaO2). Increases in neuronal activity lead to increased CMR, which is directly related to the change in CBF, a phenomenon known as “flow‐metabolism coupling” [26,28]. Flow‐metabolism coupling is a complex physiologic process involving metabolic, glial, neuronal, and vascular factors [26,28]. Local by‐products, including potassium ions, hydrogen ions, lactate, adenosine, and adenosine triphosphate modulate vascular tone and likely contribute to the mechanism of neurovascular coupling [27,29]. It is thought that neurotransmitters, especially glutamate (released with increased or excitatory neuronal activity), are the main factors augmenting activity‐induced blood flow due to the release of nitric oxide leading to cerebral vasodilation [27,28,30]. Oxygen concentration modulates the contribution of different signaling pathways, and reduced oxygen concentration can lead to adenosine release, contributing to vasodilation. Additionally, astrocytes also mediate neurovascular coupling, and nerves innervating cerebral vessels release neurotransmitters that may also be involved in this process [26,27,30,31]. The CMR can also be influenced by the functional state of the nervous system (i.e., awake versus sleep), and can be extremely high during epileptic activity, for example.
A decrease in temperature will decrease CMR and CBF in animals [32–34]. More specifically, CMR decreases by 6% per degree Celsius drop in temperature in dogs [35]. Hypothermia has been shown to have a consistently beneficial effect on neurologic outcomes in small and large animals after cardiac arrest [36]. Hyperthermia is associated with the opposite effect on CMR and CBF [35]. As such, modest hypothermia or, more importantly, the avoidance of hyperthermia may be a consideration in anesthetizing patients with conditions in which elevations of CMR or CBF may be of concern.
The cerebrovasculature is uniquely and highly sensitive to changes in PaCO2 [27,31]. An acute decrease in PaCO2 in healthy animals leads to an increase in cerebral vascular resistance and a decrease in CBF; conversely, an increase in PaCO2 has the opposite effect but is of a higher magnitude (Fig. 39.6) [31]. In monkeys, a linear relationship between both CBV and PaCO2 and CBF and PaCO2 was observed when PaCO2 ranged between 15–76 mmHg [37]. In dogs, a potential maximal cerebral vasodilatory effect occurred at between 60–70 mmHg; however, cerebrovascular responsiveness to CO2 seems to vary depending on species, experimental design, anesthetic drugs used, and CO2 levels [38]. Additionally, while a sustained decrease in PaCO2 due to prolonged hyperventilation (approximately 5–6 h) lowers CBF to some extent, it may return to baseline values over time because brain interstitial pH is one of the major regulators of CBF. Brain interstitial pH initially rises with an acute decrease in PaCO2, but then falls during sustained hyperventilation as lactate accumulates and bicarbonate falls [39–41]. As such, anesthetic management of intracranial hypertension with modest hyperventilation (discussed later) may only be employed as a relatively temporary strategy. Furthermore, because of the metabolic changes associated with a decrease in PaCO2, patients subjected to sustained hyperventilation (hypocapnia) may be at risk of developing intracranial hypertension with restoration of normocapnia as this is accompanied by CSF acidosis, significant increases in CBF, and ultimately increased ICP. Sustained hypoventilation (hypercapnia), once corrected to normocapnia, may result in a significant decrease in CBF, and the patient may, theoretically, be at risk of ischemia [26]. The change in brain extracellular pH and CBF after acute changes in PaCO2 happens because CO2 diffuses across the BBB [27,31]. However, changes in pH due to metabolic acidosis (instead of respiratory acidosis) have little effect on CBF because hydrogen ions do not cross the BBB.

Figure 39.6 Representation of the effects of arterial partial pressure of oxygen (PaO2), arterial partial pressure of carbon dioxide (PaCO2), and mean arterial blood pressure (MAP) on cerebral blood flow (CBF).
The cerebrovasculature is also sensitive to changes in PaO2 but only in hypoxic conditions (PaO2 < 60 mmHg) (Fig. 39.6), leading to cerebral vasodilation and a rapid increase in CBF (but not CMR) [31]. This sensitivity to PaO2 is dependent on the PaCO2 level. For instance, hypercapnia increases cerebrovascular sensitivity to hypoxia, and both have synergistic effects, but the vasodilatory effect of hypoxia predominates over the vasoconstrictive effect of hypocapnia [42]. Additionally, the changes in CBF due to oxygen seem to be related to oxygen content rather than oxygen tension (PaO2) because decreases in oxygen content from anemia and hemodilution also result in an increase in CBF [43,44]. The mechanism involved in hypoxia‐mediated cerebral vasodilation may involve neurogenic (neuraxial chemoreceptors) and local humoral factors [26,31].
The impact of hematocrit (i.e., blood viscosity) on CBF is controversial [45]. Anemia results in decreased cerebral vascular resistance and an increase in CBF, but as mentioned above, this response seems to be related to the decrease in oxygen content [43]. However, a study in rats demonstrated that increased viscosity decreased CBF, but only in conditions where CBF was already elevated (i.e., not in control animals) [46].
Myogenic regulation of cerebral blood flow
Myogenic regulation of CBF is related to the local vascular smooth muscle response to changes in intraluminal pressure in the cerebrovasculature. An increase in MAP leads to an increase in transmural vessel tension resulting in depolarization of vascular smooth muscle and a reduction in vascular diameter (i.e., constriction) [27]; conversely, vasodilation happens when MAP and transmural tension decrease [16]. Autoregulation of CBF is classically defined as the ability of the brain circulation to alter its resistance to maintain constant CBF within a range of MAP [47]. The lower and upper limits of CBF autoregulation likely occur at approximately 70 and 150 mmHg, respectively (Fig. 39.6). Above and below this MAP range, CBF becomes pressure‐dependent and will vary linearly with CPP [26]. There is some controversy in the literature regarding the lower limit of CBF autoregulation and values ranging from 50–70 mmHg of MAP have been suggested [27,31,48]. The initial characterization of the lower limit of CBF autoregulation at approximately 50–60 mmHg seems to originate from Lassen’s study in 1959 [47]. One complicating factor is that some authors use CPP instead of MAP on the x‐axis [31], which would naturally bring the lower limit value down because CPP = MAP – ICP. There is also significant variation between species as well as the conditions (awake versus anesthetized) and drugs used during the studies assessing the limits of cerebral autoregulation [49]; there is certainly not enough evidence to form conclusions regarding all drugs and drug combinations used clinically across all species. Furthermore, significant interindividual variation has been reported [48,49], and it is not valid to assume that autoregulation of CBF will be preserved at a MAP as low as 50 mmHg in an individual, in a broader population, or every anesthetic situation. In addition, in the normal CNS, while there is a blood flow reserve that acts as a buffer and protects against significant reductions in blood flow (i.e., hypotension), this reserve may be compromised in some clinical situations [48]. As such, defining the lower threshold of MAP below which a clinical patient is at substantial risk of ischemic injury is not easy. The authors prefer to use 70 mmHg as the lower limit of CBF autoregulation for clinical case management (Fig. 39.6).
Newer evidence suggests this topic is much more complex than commonly presented. Cerebral autoregulation does not always maintain constant perfusion through the MAP range of 70–150 mmHg, but CBF is still directly affected by changes in perfusion pressure [31]. CBF is only kept constant (i.e., the “classic” description of autoregulation) with gradual changes in blood pressure, as in nocturnal MAP changes or changes due to treatment for hypertension. For rapid changes in blood pressure (i.e., over minutes or seconds – such as those happening during surgery), CBF is more unstable and can undergo large, rapid fluctuations [27]. Slow changes in blood pressure and their effect on CBF align better with the “static autoregulation” model, whereas the impact of faster changes on CBF is more consistent with the “dynamic autoregulation” model [27,31]. Therefore, despite normal autoregulatory mechanisms, CBF may still vary with changes in blood pressure. One common misconception is that chronic hypertension leads to impairment of autoregulation (i.e., that brain vessels may become dependent on higher MAP to maintain perfusion) but treatment to lower MAP is not associated with a decrease in CBF [27].
Additionally, the effects of arterial blood gases and blood pressure are interdependent in their regulation of CBF, and it is difficult to determine the impact of each individual parameter when they change simultaneously [31]. For instance, dogs with severe hypotension showed no change in CBF following changes in PaCO2 because the vessels had already reached maximal dilation; therefore, blood pressure is a critical component of CBF [31,50].
Neurogenic regulation of cerebral blood flow
Neurogenic regulation of CBF contributes to cerebral autoregulation and can buffer surges in perfusion pressure. The cerebrovasculature is innervated by adrenergic fibers (with epinephrine, norepinephrine, dopamine, and neuropeptide Y as transmitters), cholinergic fibers (with ACh, and vasoactive intestinal peptide as transmitters), and sensory fibers (with calcitonin gene‐related peptide, substance P, and pituitary adenylate cyclase‐activating polypeptide as transmitters) as part of the sympathetic, parasympathetic, and trigeminal nervous systems, respectively [27,31]. The exact influence of cerebral vasomotor nerves is not well understood. Despite some controversies, the sympathetic nervous system generally prevents increases in CBF during resting conditions and contributes to autoregulation of CBF during rapid changes in blood pressure [27]. However, it is difficult to assess the effects of the sympathetic nervous system in isolation, as many other confounding variables are usually present. Cholinergic innervation in the brain can lead to vasodilation and further release of nitric oxide locally, but it does not seem to play a major role in autoregulation [27]. The influence of the sympathetic and parasympathetic nervous systems on CBF control seems to be species‐specific [31], so caution is advised when extrapolating across species.
Luxury perfusion
Vasomotor paralysis, which is impaired ability of vessels to respond to PaCO2 and perfusion pressure, or a loss of regional CBF autoregulation can happen in some pathological conditions leading to inadequate cerebral perfusion and hypoxia (i.e., ischemic stroke and tumor) [51–53]. Therefore, regional CBF within ischemic areas may become passively dependent on perfusion pressure, which can result in excessive blood flow relative to local metabolic demands in the face of normal or high perfusion pressure, a phenomenon called “luxury perfusion syndrome” [51,54–56]. Additionally, paradoxical flow responses can happen in some patients with these pathological conditions following an increase or decrease in PaCO2 [51,57]. In the case of decreased cerebral vascular resistance (i.e., increased PaCO2 or drugs that cause cerebral vasodilation), blood may be shunted away from areas of vasomotor paralysis to normal areas of the brain, a phenomenon called “intracerebral steal syndrome” [51]. On the other hand, an increase in cerebral vascular resistance (i.e., decreased PaCO2) may lead to blood shunting into areas of vasomotor paralysis, a phenomenon called “inverse intracerebral steal” or “Robin Hood syndrome,” which could be deleterious to normal areas of the brain [51,54].
Spinal cord physiology
The spinal cord extends rostrally from the brainstem and ends caudally within the lumbar or sacral spinal segments, depending on the species. Each segment of the spinal cord contains a pair (right and left) of spinal nerves that contain both afferent (sensory) and efferent (motor) information. Spinal cord blood flow (SCBF) is maintained via autoregulation when MAP is between 60–150 mmHg [58]. When MAP falls below 60 mmHg, SCBF is significantly reduced in dogs. Interestingly, SCBF seems to decrease with dobutamine infusions that increase MAP in healthy horses, which is concerning as low spinal cord perfusion could predispose the animal to myelopathy [59,60]. In healthy horses anesthetized with isoflurane, a significant decrease in SCBF was found with MAP levels of 100 mmHg, specifically in the thoracolumbar spinal cord region [60]. Hemodilution increases SCBF if the patient is normotensive; however, in cases of hypotension, there may be increased risk of ischemia due to limited ability to vasodilate [45]. In situations of acute blood loss representing approximately 20% of total blood volume, no changes in SCBF were detected; however, when tetracaine was administered in the subarachnoid space, a significant decrease in SCBF was detected [61].
The response of SCBF to oxygen and carbon dioxide levels is very similar to that of the brain. Hypoxia significantly increases SCBF with maximal flow reached at PaO2 levels of 30–40 mmHg [62]. Additionally, hypocapnia decreases SCBF while hypercapnia increases SCBF, with PaCO2 levels of 85 mmHg resulting in double the normocapnic SCBF [9,62].
Studies evaluating the relationship between CSF and SCBF revealed no significant change in blood flow with changes in the volume of CSF; however, elevations in CSF pressure significantly reduced SCBF [63].
Effects of vasoactive drugs, sedatives, and anesthetics
Vasoactive drugs
Most drugs used to decrease blood pressure (i.e., sodium nitroprusside and calcium channel blockers) lead to cerebral vasodilation but CBF may increase or be maintained [64–66]. The effects of catecholamine agonists and antagonists on cerebral physiology depend on baseline blood pressure, the magnitude of changes in blood pressure, autoregulation, and the status of the BBB [26]. In general, in cases where autoregulation is preserved but baseline blood pressure is outside autoregulatory limits, drug‐induced increases in blood pressure will typically increase CBF. If baseline blood pressure is within the limits of autoregulation, drug‐induced increases in blood pressure may not significantly change CBF because of activation of normal autoregulatory mechanisms [26]. There is also significant variation in drug effects on CBF (in various species) reported in the literature. Differences in study methodology undoubtedly contribute to this variation and should be taken into consideration when reviewing studies and interpreting their results.
Like anesthetics, the effect of vasoactive agents may be species‐dependent. Use of phenylephrine does not seem to change CBF or ICP in dogs but can increase CBF in rats, rabbits, and lambs [64,67–73]. Use of norepinephrine and epinephrine resulted in no significant change in CBF, ICP, and cerebral oxygen utilization, but dopamine caused an increase in all these parameters in sheep [74]. However, CBF was not changed after dopamine administration in young rabbits and monkeys [71]. Additionally, the effect of epinephrine and norepinephrine on CBF and cerebral metabolic rate of oxygen (CMRO2) was dependent on the status of the BBB in dogs, where no change was noted with an intact BBB but increases in CBF and CMRO2 were observed with increased BBB permeability [75].
The effects of β‐adrenergic agonists seem to be dose‐ and species‐dependent. At clinically relevant doses, dobutamine and dopamine do not seem to change CBF and CMRO2 in monkeys but cause a dose‐dependent decrease in CBF in dogs [76,77].
Sedatives and anesthetics
Anesthetic and sedative drugs can negatively interfere with CBF, CMR, and the electrophysiology of the brain; therefore, knowledge of these effects and how to modify them to improve clinical outcomes is crucial for better patient care. Typically, CBF and CBV vary in parallel; however, there are some clinical situations (i.e., cerebral ischemia) where they can change independently [78]. Furthermore, there are some cases where the magnitude of change in CBF may be less than that of CBV [79]. Most acute anesthetic‐induced changes in ICP occur due to changes in CBV [80]. Thus, it is important to note that anesthetic‐mediated CBF changes do not necessarily predict their impact on ICP because they may not be accompanied by similar changes in CBV [80,81]. Ideally, understanding drug‐related impacts on CPP is more important than potential isolated changes in CBF and ICP. Generally, most anesthetic drugs cause decreases in CBF and CMR, and autoregulation and CO2 responsiveness are maintained with injectable anesthetics.
Opioids
Usually, the direct effects of opioids on CBF, CMRO2, CBV, and ICP are minimal and CO2 responsiveness is typically preserved [82–84]. Most opioids cause a decrease in CBF and CBV and a decrease or no change in ICP [79,82,85–87]. However, there are conflicting results in the literature regarding the effects of some opioids, such as sufentanil and remifentanil. Some studies involving sufentanil demonstrate a transient increase in CBF and CMRO2, but minimal effects or a decrease in ICP [88]; others describe a decrease or no change in CBF and CMRO2 with no change in ICP in veterinary species [82,86]. In humans, a dose‐dependent increase in regional CBF has been shown with remifentanil [89]. The type of anesthetic protocol or the lack of general anesthesia (e.g., use of opioid alone or opioid with nitrous oxide only) may have contributed to some of this variation and, in general, sufentanil and remifentanil are not considered contraindicated in neuroanesthesia.
Nonetheless, indirect increases in ICP due to hypoventilation and hypercapnia associated with opioid administration need to be prevented in certain clinical neurological patients (i.e., traumatic brain injury and space‐occupying brain lesions). Sudden decreases in MAP that may accompany bolus doses of potent opioids in certain species should also be prevented or corrected as they may be associated with transient reductions in CPP and increases in ICP [90].
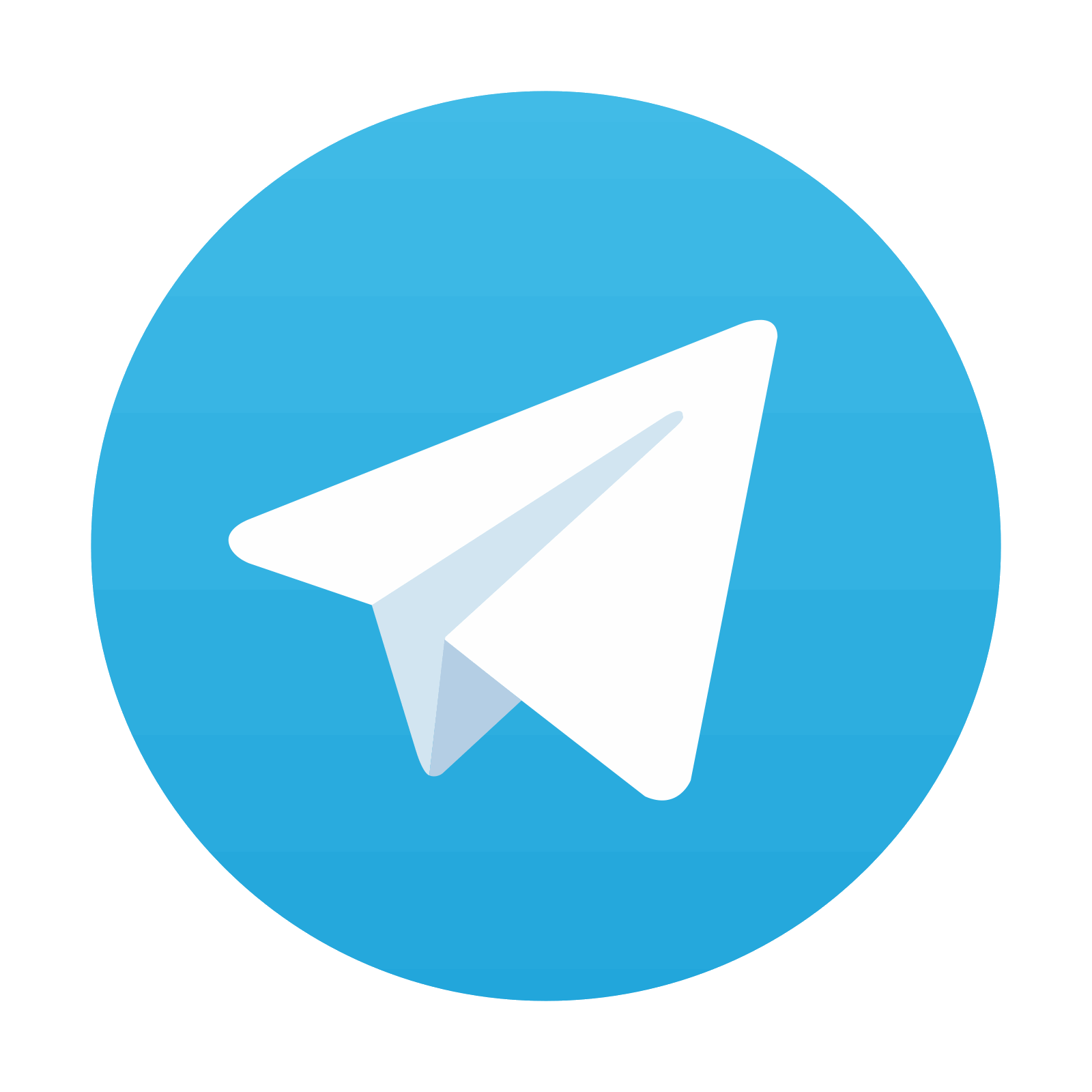
Stay updated, free articles. Join our Telegram channel
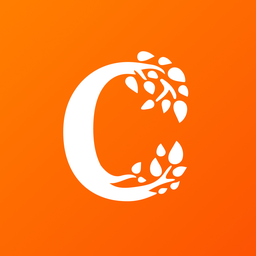
Full access? Get Clinical Tree
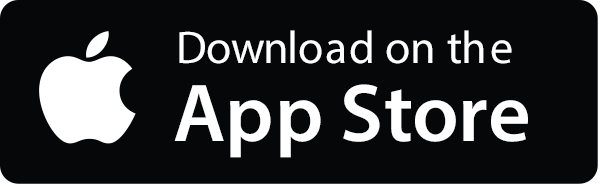
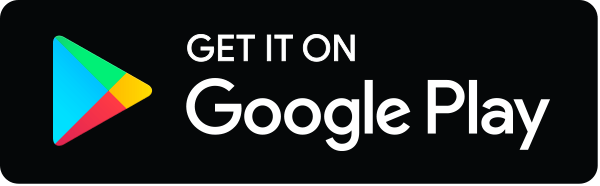