Fig. 4.1
The principle of photochemical reactions. Rose bengal, which belongs to the dye class of photosensitizers, is an efficient photosensitizer of the photodynamic type (type II), producing singlet molecular oxygen (1O2). For the photoexcitation of rose bengal, the green light absorption maximum is close to 540 nm. The singlet oxygen produced then reacts with endothelial cell components, resulting in endothelial cell injury
In collaboration with Hamamatsu Photonics K.K. (Shizuoka, Japan), the green light irradiation system, L-4887, was designed and developed by our laboratory [1]. The optical system of this apparatus is shown in Fig. 4.2. The light source is a short-arc-type super-quiet xenon lamp. The xenon lamp was selected in order to achieve the high brightness and stable light intensity necessary for irradiating a small area. The light is concentrated and prefiltered by an elliptical reflector which has a special coating to absorb infrared and ultraviolet radiation. The remaining visible light is spectrally modified by filters to produce pure green light of bandwidth 80 nm, centered at 540 nm. Elimination of infrared and ultraviolet radiations is essential, as these can heat up and damage biological tissues. The green light is then directed to a flexible optic fiber as the irradiating probe. The intensity of irradiation is controlled by an optical aperture. Alternative green light sources have also been described, e.g., a 200-fiber optic cable as green light source (530–590 nm) [35].
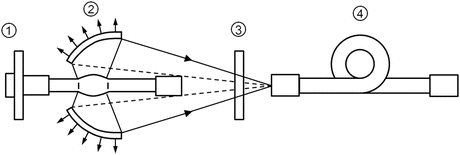
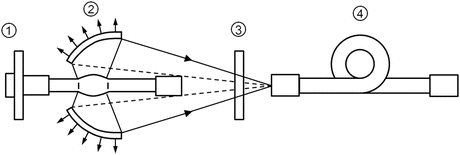
Fig. 4.2
Diagram of the optical system of L-4887, the irradiation apparatus. (1) Super-quiet xenon lamp, (2) elliptical reflector, (3) green filter with heat-absorbing capacity, and (4) flexible optic fiber. The light from the short-arc-type xenon lamp is reflected by the elliptical reflector and directed to the optic fiber via filters. Infrared and ultraviolet radiations are eliminated by the reflector and the filters. Pure green light is produced by a spectral cutoff filter. Conventional xenon lamps consume the cathode itself during prolonged use and must be replaced periodically. The super-quiet xenon lamp, however, has a special durable cathode to avoid such problems
4.2.3 Technical Aspects
Our photochemically induced thrombosis model is a modification of the technique described by Watson et al. [36] to induce focal thrombosis in cerebral vessels in the rat. In this model, a small segment of an exposed artery in an experimental animal is irradiated with green light in the presence of an intravenously administered photosensitizer such as rose bengal. Within a few minutes, the irradiated segment becomes occluded by a thrombus. We have described our approach to the femoral arteries of mice previously in great detail [12–14, 37, 38]. Briefly, animals are anesthetized with sodium pentobarbital (80 mg/kg, i.p.). A cannula is inserted into the jugular vein of each animal for injection of rose bengal. Then the right or left femoral artery is exposed, and a pulsed Doppler flow probe (PDV-20; Crystal Biotech, Hopkinton, MA, USA) is placed on it for monitoring blood flow (Fig. 4.3). Transillumination with green light (wavelength, 540 nm) is achieved using a xenon light with both a heat-absorbing filter and a green filter (model L-4887; Hamamatsu Photonics K.K., Shizuoka, Japan). Irradiation is directed via an optic fiber positioned approximately 5 mm away from a segment of the intact femoral artery proximal to the flow probe. Irradiation at an intensity of 0.9 W/cm2 is started when the baseline blood flow is stable and then rose bengal (15 mg/kg) is injected for 5 min. Irradiation is continued until the blood flow stopped. The femoral artery is considered occluded when the blood flow has stopped for more than 1 min. The time to occlusion is taken from the start of the rose bengal infusion to the cessation of blood flow (Fig. 4.4).
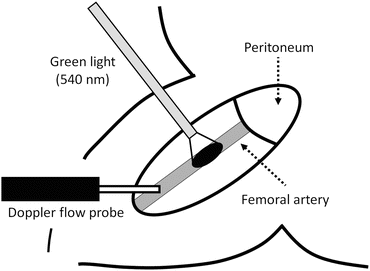
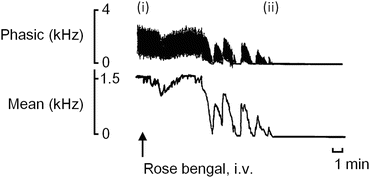
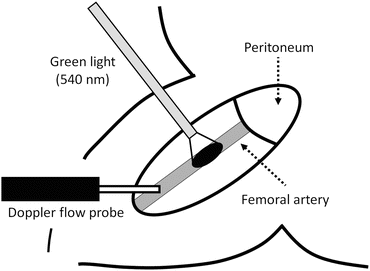
Fig. 4.3
Schematic illustration of photochemically induced thrombosis (PIT) model in the femoral artery. Either the right or left femoral artery is exposed surgically under anesthesia. Blood velocity is measured continuously by a pulsed Doppler flow probe distal to the inguinal ligament. Transillumination with green light (wavelength, 540 nm) is achieved by a xenon lamp. The light is directed by an optic fiber mounted on a micromanipulator so that its head is approximately 5 mm above the intact femoral artery, proximal to the flow probe. Rose bengal (15 mg/kg) is then injected via the contralateral femoral vein, and transillumination with green light continued until the vessel is thrombotically occluded. The femoral artery is assumed to be occluded when the blood flow has completely ceased
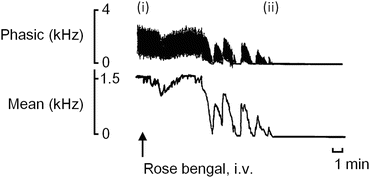
Fig. 4.4
Representative tracings of phasic and mean blood flow in a femoral artery, before and after injection of rose bengal under green light irradiation. (i) Immediately before and after injection of rose bengal 15 mg/kg, iv. (ii) Ten minutes after the initiation of the photochemical reaction. The blood flow of the irradiated artery decreased gradually with time and ceased completely about 10 min after the injection of rose bengal with green light irradiation
4.2.4 Histology of the Thrombotic Response
Light microscopic observation of the femoral artery following the cessation of blood flow reveals that the arterial lumen was occluded with a platelet-rich thrombus at the site of the photochemical reaction (irradiated segment). A representative cross section of the occluded femoral artery is shown in Fig. 4.5. The mouse femoral artery became thrombotically occluded (cessation of blood flow) about 10 min after systemic rose bengal injection and green light irradiation. Twenty-four hours later, spontaneous reflow, observed in all mice, had recovered to 86 % of the baseline level [24].
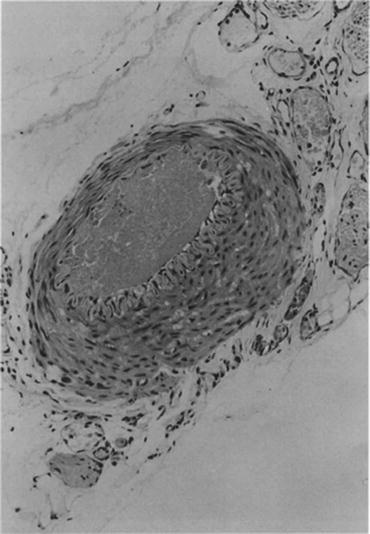
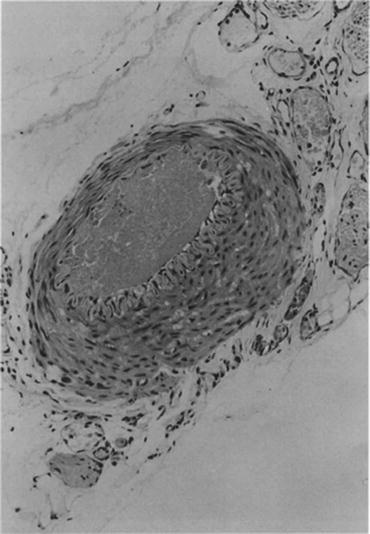
Fig. 4.5
Light microscopic view of a cross section of occluded guinea pig femoral artery which had been irradiated with green light (the site of photochemical reaction). As seen, the arterial lumen is completely occluded by a platelet-rich thrombus. The cross section is stained with hematoxylin and eosin (Reproduced with permission from Ref. [58])
The scanning electron photomicrograph in Fig. 4.6a shows a full view of the femoral artery occluded by a thrombus. Figure 4.6b is a view at higher magnification of a part of Fig. 4.6a. In this model, the thrombus was composed of distorted red blood cells and leukocytes trapped in a fibrin mesh, together with a large number of aggregated platelets (Fig. 4.6b).
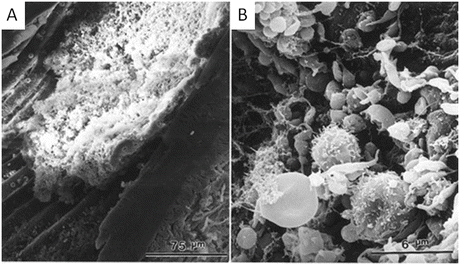
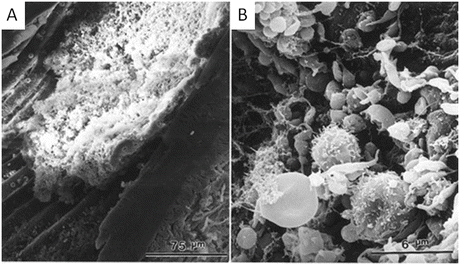
Fig. 4.6
Scanning electron photomicrographs of mouse femoral artery surface immediately after photochemically induced endothelial injury. In panels a and b, the thrombus is composed of deformed red blood cells and leukocytes trapped in a large amount of fibrin, together with numerous aggregated platelets. Panel b is a higher magnification of panel a (Reproduced with permission from Ref. [24])
4.2.5 Conclusion
This model involves a photochemical reaction between systemically administered rose bengal and transluminal green light, which cause endothelial injury followed by platelet adhesion, aggregation, and formation of a platelet- and fibrin-rich thrombus at the site of the reaction. The model represents a nonmechanical approach to induce vascular endothelial denudation. The photochemical technique is simple, fast, and reproducible. It has been used originally in guinea pigs [39–41] and rats [42–44]. Thereafter, the methodology has been adapted to induce intravascular thrombosis in mice [12–14]. However, this model can also be broadly applied to any mammalian species from mice to primates [45–47]. Thrombus formation in different species will be easily controlled by rose bengal concentration and the green light intensity. This photochemical thrombosis model has now been widely used in many kinds of genetically altered mice (reviewed in reference [48]). However, it should be mentioned that a number of transgenic mice display phenotypic variability in thrombosis severity depending on the strain background [48]. Strain-related genetic differences may also influence on the degree of photochemically induced intimal hyperplasia as discussed in Sect. 4.3. The possibility to use genetically altered animals is important, since it allows to characterize mechanisms occurring in vivo.
4.3 Photochemically Induced Arterial Intimal Thickening Model
4.3.1 Introduction
It is well known that intimal thickening occurs with the development of restenosis after percutaneous coronary intervention and the progression of atherosclerosis. To date, several experimental approaches to induce intimal thickening have been reported. These include endothelial injury induced by a flexible wire [49], a balloon catheter [50], air-drying [51], and laser injury [52]. Such injuries do lead to neointimal formation in arteries by removing the endothelium and they can be used to study atherosclerosis and restenosis after angioplasty. However, they all involve procedures that are relatively complicated and difficult to control with respect to the extent of vascular injury [53]. In the light of this situation, establishment of a simple and more reproducible animal model of intimal thickening in response to endothelial injury is very desirable. It could facilitate to gain insight into the pathophysiology of neointimal formation after vascular injury and to assess potential therapies. We have therefore adapted the thrombosis model after photochemically induced endothelial injury to induce intimal thickening originally in guinea pigs [19, 20] and rats [21–23] and thereafter in mice [24–28]. Moreover, the longer duration of these experiments may cause logistic problems, especially if therapeutic interventions should be tested. The mouse model offers an important advantage in this context.
4.3.2 Preparation of the Photochemically Induced Intimal Thickening Model
The procedure for inducing a transluminal thrombus in the mouse femoral artery is largely the same as described above. However, the main interest is focused on late sequelae of endothelial lesion and thrombosis. Therefore, the methods for assessing the outcome differ from the thrombosis model proper. The irradiated segment of the femoral artery is considered to be occluded by a platelet- and fibrin-rich thrombus when blood flow has completely stopped. Thirty minutes later the wound is closed, and the animal is returned to its cage, after it has recovered from anesthesia. Twenty-four hours later, the mice are reanesthetized, and spontaneous reflow is confirmed by monitoring the blood flow in the femoral artery. In photochemically induced thrombosis, spontaneous reflow is affected by the duration of photoirradiation, rose bengal concentration, green light intensity, and thickness of the vessel wall. Under our experimental conditions, spontaneous reflow occurred in all mice within 24 h after thrombotic occlusion of the femoral artery [24].
4.3.3 Histology on Light Microscopy
A typical pattern of intimal thickening after photochemically induced endothelial injury is shown in Fig. 4.7. Twenty-one days after the photochemical injury, an extensive neointima had formed in the subendothelial layers throughout the injured arterial segment. The neointima extended from the borders between uninjured and injured sites (positions A and E in Fig. 4.7) into the injured center (position C in Fig. 4.7). On the other hand, neointimal formation was not observed at the normal site (position F in Fig. 4.7). This process was quantified by measuring the intima-media ratio at almost equally spaced positions across the injured segment in Fig. 4.7. Position C in Fig. 4.7 had a significantly thickened neointima compared with neighboring positions [24]. Within 24 h after endothelial injury, the number of medial smooth muscle cells (SMCs) had decreased by about 28 % compared with uninjured controls, and the loss of medial SMCs was apparent in some sections after endothelial injury (Table 4.1). Seven days after injury, SMCs, which had migrated from the media, were present in the neointima and it became gradually thicker, coincident with its repopulation by cells. Neointimal formation reached a maximum of 21 days after endothelial injury and remained unchanged for as long as 42 days after the injury. Within 2 days after endothelial injury, the luminal area decreased, reflecting vasoconstriction due to the disappearance of endothelium-dependent vasodilation, but this initial decrease in the luminal area was reversed at 7 days after injury. Thereafter, with neointimal proliferation, the luminal area of the vessel decreased, but the medial area remained unchanged throughout the observation period (Table 4.1).
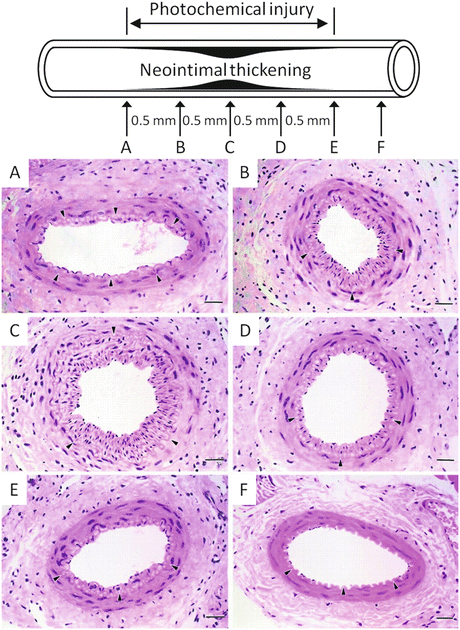
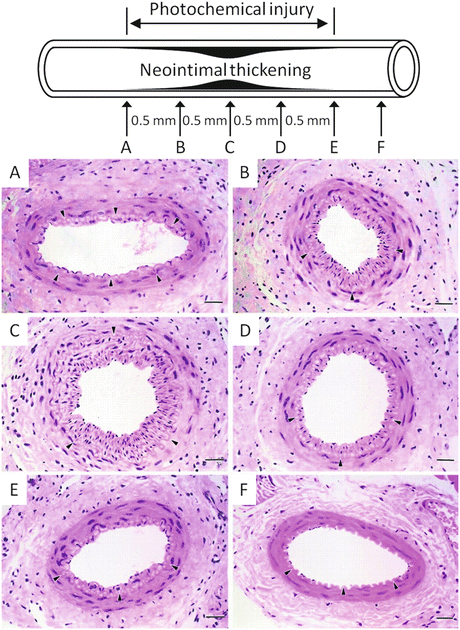
Fig. 4.7
Light photomicrographs of serial histological cross sections from mouse femoral artery 21 days after photochemical injury. a to e and f are cross sections from injured and uninjured vessels, respectively. Internal elastic lamina is indicated by arrowheads in a to f. Hematoxylin and eosin stain, bars = 50 μm (Reproduced with permission from Ref. [24])
Table 4.1
Time course of changes in intimal and medial cells; luminal, intimal, and medial areas; and intima-media ratio after photochemically induced endothelial injury
Time after injury | |||||||
---|---|---|---|---|---|---|---|
Control | 24 h | 2 days | 7 days | 14 days | 21 days | 42 days | |
Intimal cells | 0 ± 0 | 0 ± 0 | 0 ± 0 | 31 ± 5# | 70 ± 8$ | 72 ± 8$ | 83 ± 15$ |
Medial cells | 46 ± 3 | 34 ± 2 | 38 ± 5 | 44 ± 3 | 45 ± 3 | 42 ± 2 | 41 ± 3 |
Area (× 0.01 mm2) | |||||||
Luminal | 1.879 ± 0.226 | 1.519 ± 0.427 | 0.617 ± 0.093# | 1.653 ± 0.638 | 1.518 ± 0.258 | 1.204 ± 0.208 | 1.267 ± 0.166 |
Intimal | 0 ± 0 | 0 ± 0 | 0 ± 0 | 0.239 ± 0.079* | 0.301 ± 0.049# | 0.609 ± 0.079$ | 0.662 ± 0.094$ |
Medial | 0.941 ± 0.047 | 0.859 ± 0.058 | 0.935 ± 0.044 | 1.245 ± 0.079 | 1.216 ± 0.149 | 1.014 ± 0.073 | 1.136 ± 0.159 |
I/M ratio | 0 ± 0 | 0 ± 0 | 0 ± 0 | 0.180 ± 0.046 | 0.276 ± 0.058# | 0.622 ± 0.086$ | 0.661 ± 0.121$ |
n | 10 | 8 | 10 | 9 | 10 | 10 | 8 |
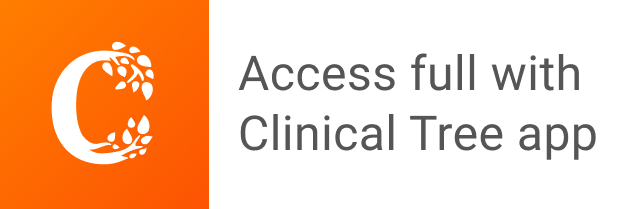