Carolyn L. Kerr Department of Clinical Studies, Ontario Veterinary College, Guelph, Ontario, N1H 2W1, Canada Alterations in body fluid volume, distribution, and composition, including disturbances in osmolality, electrolytes, and acid–base status, are not uncommon in veterinary patients presenting for anesthesia, particularly in the emergency setting. This chapter focuses on some of the most common disturbances in body fluid volume and composition, their etiologies, and the impact of their change on clinical management of patients before, during, and immediately after anesthesia. Additional literature addressing body fluid regulation physiology and pathophysiology and acid–base balance should be consulted for comprehensive reviews on this subject [1–4]. In general, perturbations in a patient’s hydration, intravascular fluid volume, electrolyte composition, and/or acid–base status may be a manifestation of a disorder that has overwhelmed the body’s ability to compensate for losses or additions in its content, or it may be indicative of a failure in regulatory mechanisms responsible for maintaining fluid and electrolyte balance. Disease processes involving the gastrointestinal, urological, and endocrine systems are the most common causes of fluid, electrolyte, and acid–base disturbances in patients requiring therapeutic interventions requiring anesthesia. A thorough patient history, physical examination, and blood electrolyte and gas analysis provide essential information during the assessment of body fluid disturbances. More in‐depth diagnostics including urine specific gravity, urine electrolyte composition, thoracic radiography, and abdominal ultrasound will be required in select cases. Consequences of abnormalities in body fluid volume and composition most commonly include alterations in central nervous system (CNS), cardiac, and neuromuscular functions, all of which may alter the patient’s response to drugs used in the perianesthetic period. Large multicenter studies have demonstrated an increase in perioperative mortality in human patients with alterations in serum electrolyte and acid–base status [5–9]. In veterinary medicine, several investigations on anesthetic‐related morbidity and mortality have shown a negative impact of increasing American Society of Anesthesiologists (ASA) status on outcome [10–13]. While underlying conditions resulting in a patient being assigned an ASA status of III and IV are likely to be associated with fluid, electrolyte, and acid–base disturbances, specific details regarding patient hydration, intravascular volume, and acid–base and electrolyte status specifically on anesthetic outcome in clinical veterinary patients remain largely unknown. Current recommendations for patient management at this time are therefore primarily based on clinician experiences, data from human trials, and experimental investigations in veterinary species rather than from prospective or retrospective studies in veterinary patient populations. In general, recommendations regarding the management of a patient’s water, electrolyte, and acid–base disturbances in the perianesthetic period are heavily influenced by the severity of the disturbance, as well as the nature of the underlying and concurrent conditions. At this time, studies evaluating patient outcome with various hemostatic alterations, as well as the response to specific goal‐directed therapies, are appearing in the literature and will help to inform future strategies, as will be discussed. In the normal animal, body fluid volume and composition, including water and electrolytes, are regulated by several overlapping mechanisms (Table 8.1). Disturbances in a patient rarely occur in isolation; however, for purposes of discussion, the chapter has been organized to address alterations in hydration, intravascular fluid volume, electrolytes, and acid–base disturbances. Body fluids are composed of water and dissolved solutes. Water makes up approximately 60% of the total body weight of domestic animal species, with two‐thirds located in the intracellular space (approximately 40% of total body weight) and one‐third located in the extracellular space (approximately 20% of total body weight). Of the extracellular water, roughly two‐thirds is in the interstitial fluid space (approximately 15% of total body weight) and one‐third in the intravascular space (approximately 5% of total body weight). Of note, the volume of water in the intravascular space constitutes approximately 50–60% of the total blood volume. In general, there are major differences in solute concentrations, notably electrolytes and negatively charged proteins, between intracellular and extracellular spaces, whereas small differences exist between the interstitial and intravascular spaces (Table 8.2). Table 8.1 Major physiologic factors that regulate body water and electrolytes [1–3]. Varying solute concentrations play a key role in the oncotic and osmotic pressures and the net electrical potential in each space. Water and solutes move between different compartments via multiple mechanisms depending on the specific substance and the barrier separating the space. A semipermeable cell membrane, which is highly permeable to water but not solutes, separates the intracellular and extracellular spaces. Water moves between these latter two spaces through the cell membrane because of an osmotic pressure gradient created by differences in the number of osmotically active particles in each space. Solute movement between the intracellular and extracellular spaces occurs through channels in the cell membrane. In some cases, solutes can flow passively through channels. However, in other cases, they require active transport. While the cell membrane separates the intracellular and extracellular spaces, the capillary endothelium separates the extracellular space into interstitial and intravascular fluid spaces. The classical description of transcapillary fluid movement is based on Starling’s principle, whereby hydrostatic and oncotic forces that exist in the capillary and interstitial fluid are the major determinants of fluid fluxes across the capillary endothelium. Essentially, the hydrostatic pressure in the capillary and the oncotic forces in the interstitium are proposed to force water out of the capillary at the arteriolar end of the capillary, while oncotic pressure in the capillary pulls fluid back into the capillary at the venular end. While this fluid movement theory has guided the basic principles of fluid therapy for many decades, recent research has shifted the understanding regarding fluid and solute movement through fenestrated capillaries. A revised Starling’s equation of fluid movement takes into consideration the endothelial glycocalyx layer, a membrane bound layer composed of glycoproteins and proteoglycans, as well as the endothelial basement membrane and the extracellular matrix [14]. The classic and revised Starling equations are illustrated in Table 8.3. Key principles in the current understanding of fluid dynamics between the interstitial and intravascular spaces in the nondisease state relevant to the veterinary clinician include: Table 8.2 Approximate electrolyte content in intracellular, interstitial, and intravascular fluid spaces [3]. Table 8.3 Classic and revised Starling’s equation [14]. In addition to fluid shifts between the various body compartments, body water intake and loss are regulated primarily by thirst and the impact of antidiuretic hormone (ADH), also known as arginine vasopressin (AVP), on the kidney. In the healthy animal, an increase in serum osmolality results in stimulation of osmoreceptors in the anterior hypothalamus, which increases thirst, and the release of AVP from the posterior pituitary. AVP, acting on collecting duct receptors of the kidney, promotes water reabsorption, resulting in a serum osmolality decrease. Alternatively, a decrease in serum osmolality results in a reduction in AVP secretion, decreasing the water reabsorption from the urine. In addition to serum osmolality, other factors can also influence AVP release from the posterior pituitary, including a decrease in blood volume or blood pressure. Several drugs, including morphine, phenothiazines, and barbiturates can also increase AVP section and alter body fluid dynamics. In humans and dogs, anesthesia and surgery increase serum AVP concentrations, a response attributed to shifts in intravascular fluid volumes and pressures [16, 17]. Although changes in serum AVP levels were not measured, urine output in dogs was reduced and total body water increased during anesthesia with routine intravenous (IV) fluid strategies using crystalloids with or without surgery [17–19]. The clinical significance of the typical body fluid increase in normal animals during anesthesia with routine fluid therapy is not known; however, the clinician should be aware that urine output alone is not a good indicator of body water volume status in the anesthetized patient. Dehydration occurs when body fluid losses are in excess of gains. Depending on the relative loss of water to electrolytes, the losses can result in hypertonic, hypotonic, or isotonic dehydration, based on the tonicity of the remaining body fluids [20]. Dehydration is typically quantified by the percent deficit of total body volume. For example, a patient is 10% dehydrated when the total body fluid deficit in liters is equivalent to [0.1] × [body weight in kg]. Clinical signs of dehydration will depend on the type of dehydration and the concurrent electrolyte changes, but, in general, signs attributed to dehydration >5% include dry mucous membranes, decreased skin elasticity, dull mentation, and increased heart rate. As the degree of dehydration progresses, the quality of peripheral pulses decreases and enophthalmos develops. With >12% dehydration, significant cardiovascular consequences including inadequate tissue perfusion secondary to intravascular volume deficits occur, and shock develops [21]. In a clinical study evaluating preoperative factors associated with hypotension in healthy dogs undergoing desexing, urine specific gravity was negatively associated with mean arterial pressure, suggesting preoperative hydration may contribute to lower blood pressure during anesthesia [22]. While there are limited large clinical trials specifically evaluating patient preoperative hydration status on outcomes in the veterinary literature, preoperative dehydration increases postoperative morbidity and mortality in large clinical trials involving humans [23]. Anesthetic drugs and ventilatory support measures may alter preload, afterload, and myocardial contractility, and therefore contribute to a significant reduction in cardiac output at surgical anesthetic planes compared to the awake state in normal veterinary patients. Fortunately, such changes are generally well tolerated in normal patients due to a substantial cardiovascular reserve. However, if a patient is dehydrated during anesthesia, the reduction in stroke volume and cardiac output will be exacerbated and the resultant cardiac output may be inadequate. In addition to addressing the underlying dehydration etiology, volume‐deficit treatment is required to improve the patient’s hemodynamic stability prior to anesthesia. As described by the Frank–Starling law of the heart, stroke volume increases with increasing end‐diastolic volume or preload within normal physiologic ranges, assuming other factors, such as myocardial contractility, remain constant. Recommended treatment regimens for fluid volume replacement generally include calculating fluid deficits, ongoing losses, and maintenance requirements. The fluid administration rate to correct dehydration should depend on severity of the patient’s clinical signs, their expected cardiovascular reserve, and urgency of the underlying condition. In the nonemergent situation, a patient’s volume deficit should be replaced over 12–24 h; if this is not possible, it is ideal if anesthesia can be delayed until the patient’s heart rate and arterial blood pressure are within normal ranges. General recommendations include replacing approximately 50% of the patient’s volume deficit over 4–6 h prior to anesthesia with the remaining deficit replaced more slowly. For the patient presenting in shock, approximately 25% of the calculated deficit should be administered rapidly, and then the patient reassessed. If the patient remains hemodynamically unstable or no improvement is noted, an additional approximately 25% of the deficit can be replaced rapidly after which an alternative strategy, such as modifying the type of resuscitation fluid, or providing additional hemodynamic support including inotropes and vasoactive agents, should be considered. An example of initial fluid therapy plans for a patient with 10% dehydration is provided in Table 8.4. Fluid choice to correct dehydration should be based on the patient’s electrolyte, acid–base, and serum protein levels; however, a balanced electrolyte solution is generally the initial fluid of choice, with adjustments made based on the patient’s response to treatment including repeated evaluation of packed cell volume (PCV), total solids, blood gases, and electrolytes. Rates up to 90 ml kg−1 h−1 in the dog or 60 ml kg−1 h−1 in the cat can be administered in an urgent setting in patients with normal cardiovascular function; however, this rate should be administered using a titrated approach whereby the patient’s hemodynamic response to 15–20 ml kg−1 (dog) or 10–15 ml kg−1 (cat) of fluid therapy is verified every 10–15 min. Rates should be reduced as soon as hemodynamic variables return to acceptable values. Patients in shock may benefit from a colloid solution if, after replacement of approximately 50% of the calculated deficits, there is no improvement of clinical signs. A colloid may also be used in conjunction with a balanced electrolyte solution, either initially or during rehydration, in patients with total solids <3.5 g dl−1 or albumin <1.5 g dl−1. Synthetic colloids, including dextrans, gelatins, and hydroxylethyl starch (HES) solutions, offer a convenient and accessible alternative to natural colloids such as fresh frozen plasma or albumin; however, synthetic products have been linked to complications including interference with coagulation, allergic reactions, and acute kidney injury in both human and veterinary patients [24–27]. Considerable geographic disparity exists regarding the availability of different products; however, current general recommendations include maintaining strict adherence to the individual product’s maximum daily dose, and avoiding their use in patients with preexisting coagulopathies, kidney disease, or sepsis. In the case of HES solutions, the maximum daily doses in dogs and cats are approximately 20 and 15 ml kg−1, respectively. In patients with specific electrolyte disturbances, details regarding recommended fluid strategies are provided in the following text. Table 8.4 Initial fluid therapy strategy in cases with varying urgency. In addition to hypovolemia due to whole body dehydration, intravascular volume deficits secondary to blood loss are not uncommon in veterinary medicine. Blood loss may occur prior to presentation to the veterinary clinic, during anesthesia and surgery, or in the postoperative period. In many cases, it is challenging to determine the quantity of blood that has been lost and the clinician must rely on a patient’s clinical signs. In the normal awake patient, an approximately 10% loss of blood volume can reduce cardiac output. However, up to a 30% blood volume loss can occur before a decrease in arterial blood pressure is observed [28]. As heart rate and blood pressure are generally the only hemodynamic variables measured in awake veterinary patients, the clinician may not recognize the severity of the vascular volume loss prior to anesthesia. In the anesthetized patient, however, hemodynamic alterations, including a decrease in blood pressure, can occur with as little as approximately 10% blood volume loss. Intraoperatively, fluid collection systems can help quantify the quantity of blood lost if volume measurements are included on the collection device (Figure 8.1). To correct for fluids used to lavage a surgical site, the PCV of the collected fluid can be measured and the quantity of blood loss calculated according to Eq. (8.1): Traditional therapeutic goals following blood loss or during active hemorrhage include maintaining indicators of perfusion, such as blood pressure, heart rate, urine output, and body temperature within normal ranges, maintaining oxygen delivery capacity by keeping the PCV above 20% (Hb >6 g dl−1), and preventing coagulopathies associated with excessive dilution of platelet and coagulation factors. Replacement of mild intravascular fluid losses with a balanced electrolyte solution is generally used in the perioperative period in patients with normal hemodynamic variables. Due to crystalloid redistribution throughout the body fluid spaces, administration volume should be approximately three times the estimated intravascular volume loss, at least initially. Administration of 15–20 ml kg−1 of a balanced electrolyte solution at a rate up to 90 ml kg−1 h−1 in the dog or 10–15 ml kg−1 of a balanced electrolyte solution at a rate up to 60 ml kg−1 h−1 in the cat can be used initially in an emergency situation with repeated volume administration based on the response to treatment; however, if a patient is hemodynamically unstable due to primary blood loss, alternatives to crystalloids should be sought. Specifically, if a patient is estimated to have lost greater than 20–25% of their blood volume, or they present with a high heart rate, low peripheral vascular pressures, and the primary cause is likely due to blood loss secondary to trauma or internal bleeding, the patient has likely lost greater than 30% of their blood volume. In such cases, colloids are highly recommended, as patient outcome may be negatively affected if fluid therapy is restricted to crystalloids [29, 30]. The individual patient and clinical situation will dictate the specific colloid required. In patients that are suitable candidates for synthetic colloids, the maximum daily dose should not be exceeded. Replacement fluids containing red cells such as packed red cells, combined with fresh frozen plasma, or fresh whole blood are indicated with major blood loss (>30%), particularly if a coagulopathy or preexisting anemia exists. In patients with uncontrolled hemorrhage, limiting the volume of crystalloid fluid administration while transitioning to fresh whole blood is currently recommended [30]. Standard transfusion practices, including crossmatching and test dosing, should be followed. While the latter recommendations provide the clinician with guidelines, the individual patient’s requirements will vary considerably and therapy should be individualized based on preexisting conditions, clinical response to treatment, and availability of suitable blood products. Use of antifibrinolytics, such as tranexamic acid, on outcomes of veterinary patients with massive blood loss remains to be determined [31, 32]. Figure 8.1 Example of a fluid collection system with numerical increments to quantify fluid/blood loss intraoperatively. In humans, both perioperative patient overhydration and underhydration lead to increased morbidity; therefore, achieving euvolemia is a critical anesthetic goal [33, 34]. Goal‐directed fluid therapy, in which individualized therapy is administered to achieve desired hemodynamic outcomes such as improvements in pulse pressure variation (PPV), stroke volume response to fluid challenge, and systolic pressure variation, is currently recommended to guide fluid therapy in humans undergoing major surgery [35, 36]. Preoperatively, patient mentation, core and peripheral body temperature, heart rate, blood pressure, urine output, and acid–base status can provide insight into rehydration adequacy in veterinary patients. Traditionally, arterial blood pressure, heart rate, urine output, repeat PCV/total solids measurement, and acid–base status have guided fluid administration rate intraoperatively. While these indices may provide an adequate guide to intravascular volume status of a patient, they are relatively nonspecific and insensitive. Ultimately, the goal is to provide intravascular volume expansion such that preload, or end‐diastolic volume, results in a desired level of cardiac output. In veterinary medicine, assessment of fluid responsiveness in mechanically ventilated canine patients using real‐time direct arterial pressure or plethysmographic (from the pulse oximeter) waveform analyses has shown promise (Figure 8.2) [37, 38]. For example, PPV ≥13%, measured from the arterial pressure waveform, reasonably predicted fluid responsiveness in approximately 83% of dogs [37]. In addition, the noninvasive plethysmograph variability index (PVI), measured from the pulse oximeter, effectively predicted the PPV threshold value with high sensitivity and specificity [37]. Figure 8.2 Example of a pulse oximeter which displays the plethysmograph variability index (PVI) used to determine fluid responsiveness in mechanically ventilated dogs. Source: Photo courtesy of Dr. R. Johnson, University of Wisconsin–Madison. For hypovolemic patients requiring anesthesia, preoxygenation for 3–5 min is highly recommended prior to anesthetic induction to compensate for a potentially reduced cardiac output and/or reduced oxygen‐carrying capacity secondary to decreased hemoglobin levels. Cardiopulmonary monitoring with an electrocardiogram (ECG), blood pressure monitor, and pulse oximeter should ideally start preoperatively and continue throughout the entire anesthetic period. Capnography and blood gas analysis should be added to the monitoring regimen during anesthesia. While no specific anesthetic regimen is contraindicated in the hypovolemic or dehydrated patient, sedative/anesthetic doses will be reduced. For example, propofol and ketamine doses, when used in combination with diazepam, were approximately 50% lower in hypovolemic compared with normal dogs [39]. In the critically ill patient, a combination of an opioid and benzodiazepine, such as hydromorphone (0.1 mg kg−1) and diazepam/midazolam (0.2 mg kg−1), is a suitable premedication and will induce less hemodynamic changes relative to other injectable techniques [39, 40]. The minimum alveolar concentration (MAC) of isoflurane is also reduced and inhalant delivery should be carefully titrated to effect [41]. The clinician should also be prepared to use constant rate infusions of opioid and nonopioid analgesics and, if feasible, locoregional blocks to reduce the inhalant anesthetic requirements and improve hemodynamic stability during anesthetic maintenance. Additional hemodynamic support measures such as inotrope and vasopressor infusions including dopamine and norepinephrine will also likely be required in these patients. Alveolar hypoventilation and the associated increase in arterial carbon dioxide (PaCO2) are not uncommon in compromised patients under general anesthesia and ventilatory support is frequently required. The increase in intrathoracic pressure during inspiration associated with positive pressure ventilation most notably reduces right ventricular preload and increases left ventricular afterload [42]. In some patients, this can result in a significant decrease in cardiac output, systemic arterial pressure (as noted with PPV and PVI), and overall oxygen delivery. The magnitude of these changes is dependent on the ventilatory strategy used and the patient’s hemodynamic status. Therefore, if ventilatory support measures are required during anesthesia of the hypovolemic patient, strategies that utilize a low tidal volume and a long expiratory pause are recommended. Most of the total body sodium is located within the extracellular space, with only low quantities located in the intracellular space, a relationship that is maintained by the cell membrane’s Na+‐K+‐ATPase pump. Dietary intake is the sodium source for the body with sodium losses primarily occurring via the kidney in the normal dog and cat. In the kidney, sodium is freely filtered at the glomeruli, with reabsorption occurring to varying degrees in the renal tubules. Aldosterone is the major endogenous substance that controls the net quantity of sodium reabsorption (Table 8.1). Factors that increase the production and release of aldosterone and, therefore, the reabsorption of sodium include angiotensin II (which is increased secondary to a decrease in renal perfusion and activation of the renin–angiotensinogen–angiotensin I pathway), hyperkalemia, and adrenocorticotropic hormone. Factors that inhibit aldosterone release include atrial natriuretic factor and dopamine. Although possessing a less significant effect than aldosterone on sodium reabsorption in the kidney, catecholamines, and angiotensin II also directly impacts sodium excretion. Specifically, catecholamines increase sodium reabsorption at the proximal tubule via alpha‐1‐adrenergic‐receptor‐mediated effects and through stimulation of renin. Angiotensin II also directly promotes renal sodium reabsorption. Serum (or plasma or whole blood) sodium concentrations are strictly a reflection of the quantity of sodium relative to the amount of water in the extracellular space and do not reflect total body sodium levels. Measured sodium levels result from factors that impact not only its own excretion and reabsorption, but also factors that influence water homeostasis in the body. Sodium, being the most plentiful ion in the extracellular fluid space, contributes most of the effective osmoles in serum. Thus, changes in serum sodium concentration impact serum osmolality and water homeostasis indirectly as described in the preceding text. The clinician needs to consider the patient’s serum osmolality, hydration, and total body water status when presented with a hyponatremic or hypernatremic patient to devise a therapeutic plan as discussed in the following text. Overall, mismanagement of patients with abnormalities in serum sodium can lead to a delayed recovery or even deleterious outcomes; clinicians are encouraged to review sodium and water regulation physiology and the management of their disturbances prior to initiating therapy. Common causes of sodium abnormalities in the dog and cat are outlined in Table 8.5. Table 8.5 Common causes of sodium disorders in dogs and cats [2, 43]. a Sodium loss is generally an isotonic sodium loss with compensatory mechanisms to restore hypovolemia, resulting in hyponatremia. Hyponatremia is generally defined as serum sodium concentrations <140 mmol l−1 in dogs, and 149 mmol l−1 in cats. Some authors define hyponatremia as a measured sodium concentration below the reference range, recognizing that values may vary according to analyzer type and nature of sample (whole blood versus serum) [43–45]. Common in hospitalized human patients, hyponatremia has been associated with an increase in morbidity and mortality, independent of the inciting cause [46, 47]. Furthermore, perioperative mortality in adult humans and pediatrics is elevated even when corrected for comorbidities [5, 6]. While no large multicenter prospective studies have been completed in veterinary medicine to date, hyponatremia has been associated with an increase in case fatality rate in both dogs and cats in a large retrospective study [48]. In veterinary patients requiring anesthesia, hyponatremia is most frequently associated with low plasma osmolality. However, it is possible for a patient to be hyponatremic and hyperosmolar if receiving mannitol or if severely hyperglycemic [48, 49]. Serum osmolality can be measured using an osmometer and it can be calculated using the following formulae (Eqs. (8.2) and (8.3)). Normally, the difference between the measured and calculated osmolality is 10 mOsm l−1. An increase in this difference reflects an increase in unmeasured osmoles, such as mannitol or ethylene glycol: or Although rare, hyponatremic patients can also have a normal osmolality if they are hyperlipemic or severely hyperproteinemic. Generally, the clinical context, including patient history, physical examination, and awareness of concurrent medication, such as diuretics and glucocorticoids, in conjunction with standard preoperative diagnostics including serum biochemistry, provides the clinician with the most likely etiology and associated osmolality of the hyponatremia. In more complex cases, or cases with moderate to severe hyponatremia, urine electrolyte content and osmolality should be measured prior to initiation of therapy. In addition to varying states of osmolality, patients can have different hydration states associated with low serum sodium levels. Hyponatremia with low plasma osmolality can be associated with hypovolemia, hypervolemia, or normovolemia, depending on the underlying etiology [44, 50]. A thorough preoperative assessment of patient hydration is also crucial to manage the patient appropriately in the perioperative period. The primary disturbances resulting in hypovolemic, hypoosmolar, hyponatremia are rarely associated with primary sodium loss; rather they are generally associated with isotonic or hypoosmolar fluid losses. Hyponatremia in these cases results from the compensatory mechanisms aimed at restoring blood volume including increased water intake and renal tubular reabsorption of water. In dogs, gastrointestinal or third‐space losses are the most common extrarenal etiologies of hyponatremia, while in cats, urologic diseases, gastrointestinal, and third‐space losses are the most common (Table 8.5) [48]. Clinical signs associated with hyponatremia are relatively nonspecific. Gastrointestinal signs such as vomiting and diarrhea are not uncommon and CNS signs can develop as a result of water shifting from the extracellular to the intracellular space due to the osmolality gradient. Neurons can compensate if the changes occur slowly by shifting other solutes out of the cell to minimize water accumulation. However, if the changes occur rapidly or are excessive, CNS signs such as lethargy, disorientation, ataxia, coma, and seizures can develop. Clinical signs of CNS dysfunction tend to occur with sodium levels <120 mmol l−1 or if the rate of decrease is >0.5 mmol l−1 h−1. Unfortunately, clinical signs associated with hyponatremia or excessive correction rates will be masked with sedation and anesthesia; therefore, the anesthetist will need to rely on repeat serum sodium measurements to guide ongoing therapy if the condition is not corrected fully prior to anesthesia. The major concerns in the perioperative period for patients with hyponatremia relate to effects on the patient’s CNS and volume status. The therapeutic approach before anesthesia depends on clinical sign severity and disorder chronicity. If possible, even mild hyponatremia and volume disturbances should be corrected prior to anesthesia based on the current association of hyponatremia with poor outcomes. The patient’s clinical signs and disease chronicity should guide the rate and volume of IV therapy. Ideally, treatment of the primary cause can be initiated in addition to correction of the electrolyte disturbances. For example, patients with hypoadrenocorticism should receive mineralocorticoid replacement therapy in addition to fluid therapy to correct electrolyte disturbances prior to anesthesia. In urgent situations, correction of the hyponatremia should be started prior to anesthesia and continued throughout the anesthetic period and into recovery. The optimal treatment regimen for hyponatremic veterinary patients has yet to be determined; however, experts in critical care and internal medicine report consistent recommendations regarding the rate of change in serum sodium concentrations as discussed in the following text. In the “severely symptomatic hypoosmolar hyponatremic patient” (for example, presenting with seizures or markedly abnormal behavior), treatment with small boluses (2 ml kg−1) of 3% NaCl to increase serum sodium by 4–6 mmol l−1 is recommended [43]. Improvement in clinical signs attributed to the hyponatremia occurs when serum sodium reaches 125 mmol l−1, at which point treatment should be adjusted according to the chronicity of the disease and the patient’s overall volume status as described in the following text. In the “acutely (<48 h) dehydrated hypovolemic hyponatremic” veterinary patient requiring anesthesia, administration of a balanced electrolyte solution such as lactated Ringer’s solution, Plasma‐Lyte A, Plasma‐Lyte 148, or Normosol‐R, to replace volume deficits, is recommended [44]. Correction of the volume deficit generally resolves the hyponatremia and volume replacement can occur relatively rapidly to reestablish extracellular fluid status. Changes in patient serum sodium concentrations at a rate of 1–2 mmol l−1 h−1 are considered acceptable. In the “hypovolemic dehydrated” patient with “chronic hyponatremia,” serum sodium should be increased by 0.5 mmol l–1 h–1 or 10–12 mmol l−1 d−1 at maximum to avoid the risk of adverse neurological outcomes, including intracranial hemorrhage or osmotic demyelination [51–54]. Careful consideration of the patient’s measured or calculated osmolality with consideration of the impact of other solutes such as glucose is required before initiation of treatment. For most cases, a balanced electrolyte solution is recommended to correct the hypovolemia with serial hourly serum sodium measurements used to guide fluid administration rate. Differences in sodium and osmolality exist among the various isotonic balanced electrolyte solutions. Of the balanced electrolyte solutions, lactated Ringer’s solution is preferable for patients with chronic hyponatremia, as it has a lower osmolality and sodium content relative to the other solutions in this category. If the patient’s rate of serum sodium increase is excessive following therapy initiation with a balanced electrolyte solution, further assessment of the patient’s sodium deficit and infusion rate for correction is required. It is recommended that further resources with an in‐depth discussion related to the treatment of hyponatremia are consulted in the nonurgent situation [44]. In the “normovolemic” or “hypervolemic chronically hyponatremic” patient who requires anesthesia, management will be heavily dependent on the underlying cause of the electrolyte disturbance and the nature and severity of the symptoms associated with hyponatremia. In some cases, individualized fluid therapy administration, using a variety of tailor‐made fluids and possibly diuretic administration, will be necessary to correct both the sodium deficit and hypervolemic state [44]. Serum hypoosmolality has been shown to decrease halothane MAC in dogs [55]. Although the impact of hyponatremia on other anesthetic agents has not been determined, the clinician should select agents that can be titrated to effect to avoid overdose and adverse hemodynamic consequences. Intraoperatively, in patients with the potential for significant intravascular volume losses during surgery, a separate colloid infusion may be considered to replace intraoperative intravascular losses. If a synthetic colloid is used, selection of a product with sodium levels close to physiologic values should avoid excessive change in sodium concentration. As hyponatremic patients, particularly those with intravascular volume deficits, may also be prone to hypotension, the anesthetist should be prepared to use vasopressors and/or inotropes during anesthesia. Serum sodium and hydration status monitoring should continue during anesthesia and into the postoperative period. The syndrome of inappropriate antidiuresis or syndrome of inappropriate antidiuretic hormone (SIADH) secretion is not uncommon in critically ill human patients and may occur in the postoperative period. The condition, although rare, has been reported in both dogs and cats [44]. A decreased urine output despite normovolemia should trigger further diagnostic investigation to prevent iatrogenic hypervolemia from excessive fluid administration. Hypernatremia is defined as serum sodium concentrations >160 mmol l−1 in dogs, and 175 mmol l−1 in cats. Hypernatremia is always associated with hyperosmolality. It is relatively rare in patients with normal mentation and free access to water but can be observed in the perioperative period in critically ill patients. As with hyponatremia, patients can be hypovolemic, normovolemic, or hypervolemic depending on the primary cause (Table 8.5). In one large retrospective study, gastrointestinal fluid losses, losses associated with diabetes insipidus, and fever were the most common pathophysiologic factors associated with hypernatremia in dogs, while in cats, kidney disease (acute and chronic) and gastrointestinal factors were the most common [56]. In this same study, the primary conditions resulting in hypernatremia were neurological and neoplasia in dogs and urological and neurological in cats. In terms of potential iatrogenic‐acquired hypernatremia, hypernatremia associated with hypertonic saline or sodium bicarbonate administration is generally transient in the awake patient if unrestricted water intake is possible or if it is followed with appropriate balanced electrolyte solutions in the anesthetized patient. A recent study demonstrated hypernatremia following a single dose of activated‐charcoal‐containing sorbitol in dogs, a result believed to be secondary to loss of water via the gastrointestinal tract [57]. Clinical signs associated with hypernatremia generally occur with serum sodium levels exceeding 170 mmol l−1 and include anorexia, lethargy, vomiting, muscular weakness, disorientation, ataxia, seizures, coma, and death. If patients have concurrent hypovolemia or hypervolemia, they may show cardiovascular changes such as tachycardia or pulmonary edema, respectively. As with hyponatremia, hypernatremia should be corrected slowly over at least 48 h. If the patient is acutely (<48 h) hypernatremic and hypovolemic, the plasma volume deficit can be replaced with a balanced electrolyte solution over several hours followed by a slower correction of the hyperosmolality with the administration of hypotonic IV solutions such as 0.45% saline or 5% dextrose [44]. In cases of chronic hypernatremia, the serum sodium concentration should be decreased by no more than 1.0 mmol l−1 h−1 due to the risk of neurologic complications. In cases of hypervolemia, loop diuretic administration may facilitate sodium excretion; however, if renal dysfunction is present, alternative therapies such as hemodialysis will be required. During the anesthetic period, the clinician should continue to correct the hypernatremia as described previously. In the experimental setting, hypernatremia is associated with an increase in the MAC of inhalant anesthetics; it is possible that there will be increased inhalant anesthetic requirements [55, 58]. As discussed previously, a patient’s intravascular volume status will also likely affect anesthetic requirements, and anesthetic depth should be monitored and delivery of anesthetics adjusted based on the basis of the individual animal assessment. Chloride is the major anion in the extracellular fluid and contributes significantly to maintaining extracellular osmolality. Intracellular chloride concentrations vary depending on the cell type; however, intracellular concentrations are consistently considerably lower than plasma levels. As with sodium, dietary intake is the main chloride source for the body with losses primarily occurring via the kidney and gastrointestinal tract. Plasma chloride regulation occurs in the kidney with acid–base and sodium concentrations being the major factors that influence chloride reabsorption or excretion [59]. Alterations in serum chloride may reflect a primary disorder of chloride, sodium, or total body water balance. Alternatively, they may be the consequence of a compensatory response in a patient with primary changes in acid–base status (see the section titled “Acid–Base Homeostasis and Disturbances”). Common causes of abnormalities in serum chloride in the dog and cat are outlined in Table 8.6. Clinical signs related to serum chloride alterations are generally nonspecific and are associated with the concurrent alterations in osmolality/total body water or acid–base status. As such, consideration of these factors is required for appropriate patient management. To correct for disturbances in total body water balance on measured plasma chloride, the effect of body water changes on serum chloride should first be determined by calculating a corrected chloride concentration. This can be achieved by using measured sodium relative to normal sodium concentrations as a correction factor according to the following equations (Eqs. (8.4) and (8.5)). If the corrected chloride values fall within normal values, the primary etiology of the abnormal measured chloride reflects abnormalities in total body water, and therapy should be directed accordingly:
8
Perioperative Fluid, Electrolyte, and Acid–Base Disturbances
Introduction
Body Fluids
Variable
Regulatory mechanism
Primary stimulus
Primary effect
Water
ADH
Serum osmolality
Increase water reabsorption in kidney
Thirst
Serum osmolality
Increase water intake
Sodium
Aldosterone
Hyperkalemia, angiotensin II, adrenocorticotropic hormone (ACTH)
Increase Na+ reabsorption in kidney
Epinephrine
Sympathetic nervous system stimulation
Increase Na+ reabsorption in kidney
Angiotensin II
Renal perfusion
Increase Na+ reabsorption in kidney
ADH
Serum osmolality
Alter water reabsorption in kidney
Chloride
Kidney
Sodium, acid–base status
Alter sodium and chloride reabsorption
Potassium
Aldosterone
Hyperkalemia, angiotensin II
Decrease K+ reabsorption in kidney
Epinephrine
Sympathetic nervous system stimulation
Increase intracellular K+ movement
Insulin
Serum glucose
Increase intracellular K+ movement
Calcium
Parathyroid hormone
Serum‐ionized calcium
Increase Ca2+ reabsorption in kidney
Increase Ca2+ mobilization from bone
Increase calcitriol synthesis
Calcitriol
Parathyroid hormone
Increase Ca2+ intestinal absorption
Calcitonin
Serum‐ionized calcium
Reduce Ca2+ mobilization from bone
Intracellular fluid (skeletal muscle cell; mmol l−1)
Interstitial fluid (mmol l−1)
Intravascular fluid (plasma; mmol l−1)
Na+
12
145
142
K+
140
4
4.3
Ca2+
4.0
2.4
2.5
Mg2+
34
1.1
1.1
Cl−
4
117
104
HCO3 −
12
27
24
HPO4 2−, H2PO4 −
40
2
2
Proteins−
50
0
14
Classic Starling equation
Revised Starling equation
Net filtration = KfS [(Pcap − Pi) − σ (πcap − πi)]
Net filtration = KfS [(Pcap − Psg) − σ (πcap − πsg)]
KfS = filtration coefficient
Pcap = capillary hydrostatic pressure
Pi = interstitial hydrostatic pressure
πcap = capillary oncotic pressure
πi = interstitial oncotic pressure
KfS = filtration coefficient
Pcap = capillary hydrostatic pressure
Psg = sub‐glycocalyx hydrostatic pressure
πcap = capillary oncotic pressure
πsg = sub‐glycocalyx oncotic pressure
Dehydration and Intravascular Volume Deficits
3‐year‐old Labrador Retriever
3‐year‐old Labrador Retriever
Case urgency
High: scheduled for surgery in 4 h
Low: scheduled for surgery in 12–24 h
% dehydration
8%
8%
Patient weight
30 kg
30 kg
Volume deficit
= 0.08 × 30 kg = 2.4 l
= 0.08 × 30 kg = 2.4 l
Intravenous fluid
Balanced electrolyte solution
Balanced electrolyte solution
Initial fluid plan
Replace 50% of deficit over 4 h = 300 ml h−1 for 4 h
Replace remaining deficit related to dehydration over next 8 h = 150 ml h−1 for 8 h
Maintenance fluid requirements and ongoing losses should be added to the volume calculated for dehydration correction
Replace 100% of deficit over 12 h = 200 ml h−1 for 12 h
Maintenance fluid requirements and ongoing losses should be added to the volume calculated for dehydration correction
Electrolytes
Sodium
Hyponatremia
Hypernatremia
Altered losses
Increased Na + loss a
Gastrointestinal loss (hypovolemic, hypoosmolality)
Renal loss (hypovolemic, hypoosmolality)
Vascular loss (hypovolemic, hypoosmolality)
Decreased water loss (gain of free water)
Drugs (hypervolemic, hypoosmolality)
Congestive heart failure (hypervolemic, hypoosmolality)
Liver disease (hypervolemic, hypoosmolality)
Renal disease (hypervolemic, hypoosmolality)
Psychogenic polydipsia (normovolemia, hypoosmolality)
Syndrome of inappropriate antidiuretic hormone secretion (normovolemia, hypoosmolality)
Increased water loss relative to Na +
Gastrointestinal loss (hypovolemic)
Renal loss (hypovolemic or normovolemic)
Cutaneous loss (hypovolemic)
Respiratory loss (hypovolemic)
Fever (hypovolemic)
Decreased Na + loss
Hyperadrenocorticism (hypervolemic)
Translocation
Greater Na + movement relative to water
Third‐space loss (hypovolemic, hypoosmolality)
Movement of water from intracellular fluid (ICF) into extracellular fluid (ECF)
Solute addition to ECF (hyperosmolality)
Greater water movement relative to Na +
Third‐space loss (hypovolemic)
Altered intake or input
Decreased intake
Rare situation
Decreased water intake
Water deprivation (hypovolemic)
Increased Na + intake
Salt poisoning (hypervolemic)
Hypertonic fluid or Na + bicarbonate administration
Hyponatremia
Hypernatremia
Chloride
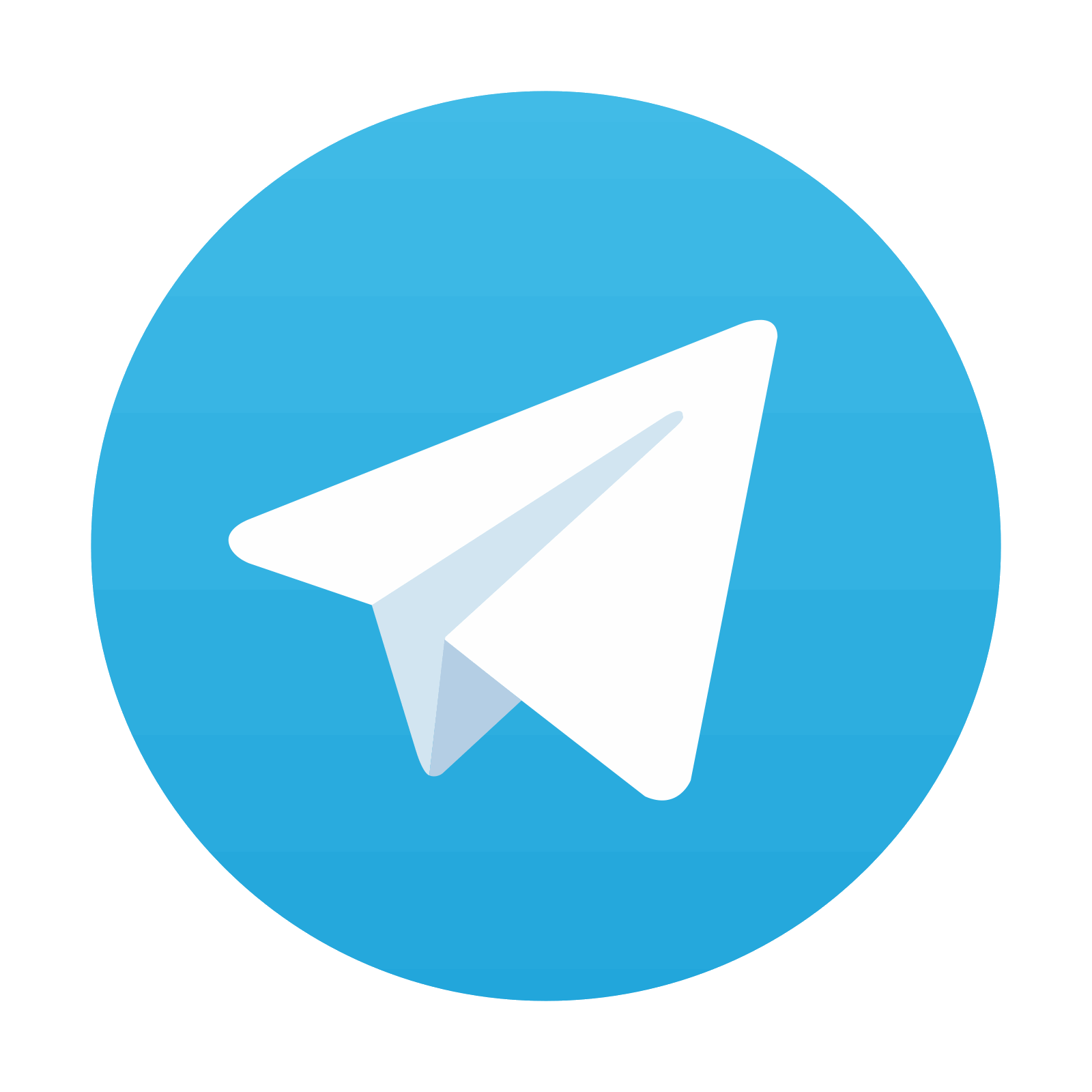
Stay updated, free articles. Join our Telegram channel
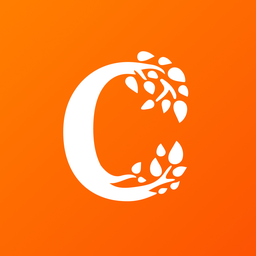
Full access? Get Clinical Tree
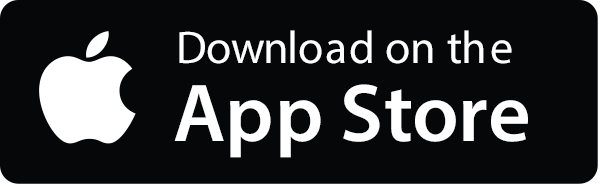
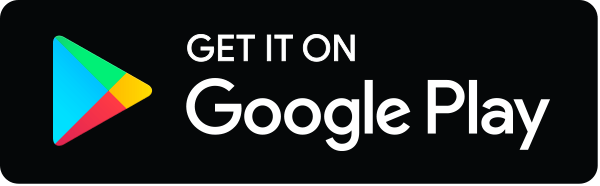