Introduction
The purpose of anesthesia is to provide a reversible alteration in central nervous system (C NS) function characterized by unconsciousness, amnesia, analgesia, and immobility suitable for completion of a wide variety of procedures including invasive surgery. Unfortunately, the drugs needed to produce these changes in CNS function also compromise patient homeostasis. Thus, anesthetic management can be considered a balancing act whereby adequate anesthetic depth is weighed against depression of other key organ systems. The ability to monitor the function of both the CNS and these other organs, notably the cardiovascular and pulmonary systems, is crucial in maintaining this balance.
Statistics regarding anesthetic risk, morbidity, and mortality in dogs and cats are presented in Chapter 1. The traditional method for measuring anesthetic outcomes in veterinary medicine has been anesthetic mortality. While mortality clearly fits the definition of an adverse anesthetic outcome, lack of mortality is, at best, a very crude benchmark for defining a successful outcome. Instead, as the standard of veterinary care continues to advance, there is an increasing movement to focus on more suitable measures of anesthetic morbidity. By striving to minimize morbidity in the perianesthetic period, the quality of care can be substantially improved and mortality rates will inevitably fall.
This approach is predicated on the ability to define and identify key pathophysiological abnormalities that contribute to anesthetic morbidity, such as hypotension, hypoxemia, hypercapnia, and hypothermia, and to institute corrective measures and/or supportive care. In order to do this effectively, personnel specifically trained in anesthetic monitoring and appropriate monitoring equipment are required. For more information on the American College of Veterinary Anesthesiologists Recommendations for Monitoring Anesthetized Patients, please visit www.acva.org.
In general, the transition from a light to a deep plane of anesthesia is associated with the following: loss of recall, loss of awareness, lack of movement in response to a noxious stimulus, and finally lack of a hemodynamic or electroencephalographic (EEG) response to a noxious stimulus. Anesthetic depth represents the balance between the administered doses of anesthetic and adjuvant drugs, the intensity of surgical stimulation (which tends to awaken patients), and the severity of illness (which tends to augment anesthesia). For longer procedures, anesthetic requirements tend to decrease over time within a single anesthetic episode. In these situations, it is appropriate to make repeated attempts to incrementally decrease the amount of anesthetic administered and observe the patient’s response.
Subjective methods
The physical signs of anesthetic depth depend largely on evaluation of muscular tone and reflex activity. In dogs and cats, these will vary from moment to moment, from individual to individual, and between anesthetic drugs. Since no one sign alone defines anesthetic depth, it is important to assess as many signs as possible and integrate the information they provide.
The following are key points for evaluation of the physical signs of anesthetic depth:
(1) Careful selection of administered doses of injectable and inhalant anesthetic drugs is mandatory, but in no way guarantees an appropriate depth of anesthesia will be achieved. Measurement of end-tidal inhalant anesthetic concentration (ETagent) is a relatively new monitoring modality in veterinary clinical anesthesia that measures the amount of anesthetic in the patient. It is analogous to measuring plasma concentrations of injectable drugs but is clinically applicable as it provides continuous, real-time data to the anesthetist. While ETagent is not a measure of anesthetic depth, it does provide more insight than vaporizer setting, which simply estimates the amount of drug delivered to the breathing circuit.
(2) Spontaneous movement is a reliable sign of a light level of anesthesia with most anesthetics, though there are several exceptions to this rule. Focal myoclonus (muscle twitching) has been associated with etomidate and propofol administration and should not be interpreted as a light level of anesthesia. Spontaneous muscular movement is common with opioid-based anesthetic protocols and should also not be interpreted to indicate a light level. Muscle hypertonus can be a feature of ketamine-based protocols and should not be interpreted as a light level of anesthesia.
(3) Reflex movement in response to surgical stimulation is a reliable sign of a light level of anesthesia. It does not, however, mean that an animal is experiencing pain from a noxious stimulus.
(4) An abrupt increase in heart rate (HR), arterial blood pressure (ABP), respiratory rate (RR), or tidal volume (TV), specifically in response to surgical stimulation, is generally considered to be a reliable sign of a light level of anesthesia. In general, these physiological parameters tend to trend upward as an animal becomes more lightly anesthetized and downward with deeper anesthesia. These are not, however, reliable premonitory indicators of anesthetic depth. In some situations, physiological parameters may remain relatively stable until after an animal abruptly awakens or suffers cardiovascular collapse. There are also numerous confounding factors that will impact these parameters, in either direction, that have nothing to do with anesthetic depth. Inappropriate anesthetic depth is only one differential to consider when an animal exhibits increases or decreases in HR, ABP, RR, and/or TV.
(5) Mandibular muscle tone (“jaw tone”) should be substantial, moderate, and none at light, medium, and deep levels of anesthesia, respectively. The definitions of substantial, moderate, and none are relative and will vary in dogs versus cats, and in small versus large breed dogs. Mandibular muscle tone is assessed by the resistance encountered when trying to open the mandible. In neonatal puppies and kittens, mandibular muscle tone is usually minimal and this parameter is less useful in these patients.
(6) A change to an abdominal (diaphragmatic) breathing pattern usually signals a deeper level of anesthesia. This will be accompanied by changes in physiological parameters including decreased RR and TV.
(7) The presence of a palpebral reflex is a reliable indicator of a light level of anesthesia in most patients. The absence of it suggests a medium or deep level. Some individuals, however, fail to exhibit a palpebral reflex even though their anesthesia level is light. With ketamine use, the palpebral reflex is always present so this parameter is not a reliable indicator of anesthetic level in this situation.
(8) The presence of a pupillary light reflex (pupillary constriction in response to a bright light directed onto the retina) and the presence of a dazzle reflex (a blink in response to a bright light) are reliable indicators of a light to light-medium level of anesthesia. The pupillary light reflex may be minimized or eliminated by administration of anticholinergics (atropine or glycopyrrolate) so this parameter is not a reliable indicator of anesthetic level in this situation.
(9) The eyeball position is central (and the pupil size is medium) when the animal’s anesthesia level is light, is rotated ventromedially when the level is medium, and is central again (and the pupil is dilated) when the level is deep. The eyeball does not rotate when ketamine is used so this parameter is not a reliable indicator of anesthetic level in this situation. Nystagmus does not normally occur in anesthetized dogs and cats at any depth.
(10) The gag and swallow reflexes are reliable indicators of a light level of anesthesia in virtually all circumstances in anesthetized dogs and cats.
Objective methods
Neurophysiological monitoring as a tool to quantify anesthetic depth is not a new field of study. One type of neurophysiological monitoring, EEG analysis, involves interpretation of brain waves recorded from the scalp during anesthetic delivery, which exhibit characteristic changes during the transition from light to medium to deep levels of anesthesia. In some cases, the raw EEG is studied directly or alternatively, EEG derivatives such as frequency or power distribution, amplitude, or correlations between recorded signals are analyzed. Unfortunately, EEG signal and pattern analysis have proved to be complex and difficult to accomplish in real time, making this approach impractical in a clinical setting.
Another approach to neurophysiological monitoring involves evoked potential (EP) studies. Both auditory (brain stem recordings in response to an auditory stimulus) and somatosensory (brain stem and cortical recordings in response to electrical stimulation of a peripheral nerve) EPs show progressive changes with alterations in anesthetic depth. This approach suffers from many of the same disadvantages as analysis of spontaneous EEG potentials and is not useful as a real-time monitor of anesthetic depth clinically.
The Bispectral Index™ (BIS) Monitoring System (Covidien-Nellcor, Boulder, CO) was introduced in 1994 as a novel measure of the level of consciousness and is based on an algorithmic analysis of a patient’s EEG during anesthesia. BIS is a statistically based, empirically derived parameter based on the weighted sum of selected EEG subparameters. It includes a time domain, a frequency domain, and other high-order spectral variables. Essentially, the BIS monitor takes a complex signal (the EEG), analyzes it, and processes the result into a single dimensionless number between 0 (equivalent to EEG silence) and 100 (equivalent to awake and alert). The proprietary calculation algorithm used was based on the evaluation of numerous EEG recordings taken from healthy human volunteers at specific clinically important end points and plasma anesthetic drug concentrations. While the calculation is extremely complex, the more recent availability of cost-effective, rapid, powerful computer processors has facilitated clinical introduction of this technology and others like it.
Clinically, the BIS monitor displays a value that changes, with a minimal lag time, along with changes in anesthetic depth. In general, BIS values above 90 are compatible with an awake and alert state, 80–90 with anxiolysis, 60–80 with hypnotic or moderate obtundation, below 60 with loss of recall, below 50 with unresponsiveness to verbal stimuli, below 20 with burst suppression, and 0 with cortical silence (isoelectric EEG). Not all anesthetics affect BIS in the same way. Propofol, midazolam, and thiopental strongly depress it, inhalational anesthetics have an intermediate effect, opioids have little effect, and nitrous oxide and ketamine tend to increase the BIS value. BIS does not monitor analgesia and may not predict reflex movement or hemodynamic activity in response to noxious stimulation. The recent introduction of other systems also based on calculated EEG indices, such as the Narcotrend™ Monitor (Arbeitsgruppe Informatik/Biometrie der Anästhesie, Hannover, Germany) and the Cerebral State™ Monitor (danmeter, DK-5000 Odense C, Denmark), appear to perform similarly to the BIS monitor.
While the use of BIS and other similar monitors continues to increase in human operating rooms worldwide, it is important to note that they have not replaced physical evaluation of patients and it is not likely that they ever will. The benefits of quantitative monitoring of anesthetic depth include minimizing the risk of patient awareness, reduction of anesthetic drug dosages, and shortened recovery times. While these tools are currently being used by veterinary anesthesia specialists in academic and private practice settings, their potential place in general practice remains to be determined.
Physiological consequences of the anesthesia
Although the specific pharmacodynamic effects of the various anesthetic drugs vary, the mechanisms by which they contribute to morbidity and mortality are similar. Excessive bradycardia, dysrhythmias, myocardial depression, vasodilation, hypotension, hypoventilation, hypoxemia, and hypothermia are among the most common examples. Since all of these (except hypothermia) can be classified as derangements in cardiopulmonary function and all may negatively impact other organ systems, perianesthetic monitoring tends to prioritize cardiovascular and pulmonary stability.
These two systems function, in a very broad sense, to deliver oxygen (O2) and remove carbon dioxide (CO2) from tissues. Oxygen delivery (DO2) involves the pulmonary system, which is responsible for loading arterial blood with O2 (i.e., oxygenation), and the cardiovascular system, which pumps oxygenated blood to tissues (i.e., circulation). Carbon dioxide removal is also accomplished by the lungs through atmospheric gas exchange (i.e., ventilation). It is useful to consider this big picture when monitoring individual patient parameters and always strive to put a particular measurement or trend into context. Figure 6.1 is a simplified illustration of the key factors determining circulation and oxygenation, including their relationships to each other and to O2 delivery. Figure 6.2 illustrates the key factors determining ventilation and how they relate to CO2 removal.
DO2 is the product of cardiac output (CO) and arterial oxygen content (CaO2) as shown below. Reported DO2 values are indexed to body surface area (i.e., milliliter of O2 per minute per square meter) to facilitate comparisons as CO will vary depending on patient size. Estimates for normal dogs are approximately 800 mL/min/m2 (or about 25–35mL/min/kg).
Note that CaO2 represents the total amount of oxygen present in blood and is a function of arterial O2 saturation (defined as the percent of O2-bound hemoglobin binding sites to total hemoglobin binding sites; SaO2), the concentration of hemoglobin and, to a minor extent, the amount of oxygen dissolved in blood (defined as the arterial partial pressure of oxygen; PaO2). Normal CaO2 values are approximately 20 mL of O2 per deciliter of blood based on the following formula:
Based on these equations, a decrease in CaO2 does not necessarily result in a decrease in DO2 if CO is augmented. In human critical care, there has been a movement toward perioperative “targeted oxygen delivery” or “oxygen delivery optimization” in high-risk patients as a means of improving outcomes. Whether such strategies are actually effective on a population basis remains controversial and further work is needed to determine the appropriate end points and timing of interventions. Because calculation of DO2 requires measurement of CO, SaO2, hemoglobin, and PaO2, it is infrequently used during veterinary clinical anesthesia monitoring.
Oxygen consumption (VO2) is the amount of oxygen consumed by tissues each minute and can be calculated by multiplying the difference between CaO2 and mixed venous oxygen content (CvO2) by CO as shown below. Estimates of VO2 in normal dogs are approximately 150mL/min/m2 (or about 4–10mL/min/kg).
VO2 is not normally limited by DO2 as tissues can extract a greater proportion of oxygen from arterial blood (i.e., the CaO2 – CvO2 difference increases) in the face of lowered CO. As CO decreases to critically low values, however, VO2 becomes DO2 (or flow) dependent.
The oxygen extraction ratio (OER) is the proportion of O2 consumed to O2 delivered as shown below:
Because CO appears in both the numerator and denominator and because hemoglobin is assumed to be the same in both arterial and venous blood, the above equation can be simplified to include only saturation instead of oxygen content. The simplified equation is shown below:
For optimal accuracy, this equation requires measurement of SaO2 and SvO2 using cooximetry to analyze arterial and mixed venous blood samples drawn from the patient. While an estimate of SaO2 is routinely calculated and reported by commercial blood gas analyzers using the measured parameters (pH, PO2, and PCO2), an arbitrary default hemoglobin concentration, and an algorithm based on the oxyhemoglobin dissociation curve, this method is less accurate than co-oximetry. Since SvO2 values fall on the steep part of the dissociation curve, estimation of SvO2 using the blood gas analyzer method is associated with an even greater margin or error. The fact that the oxyhemoglobin dissociation curve used by commercial blood gas analyzers is based on data from normal human adults introduces further potential error when extrapolating to dogs and cats.
The OER is a clinically useful method for assessing the adequacy of CO without actually measuring it (though it does require mixed venous sampling). Its primary utility is in critical care settings as opposed to clinical anesthesia monitoring. Under normal resting physiological conditions OER is approximately 0.25 and ratios >0.4 suggest serious impairment of CO.
Monitoring circulation, oxygenation, and ventilation
While measurement (or at least estimation) of DO2 and OER may be indicated in critically ill veterinary patients requiring intensive care, they are not practical in the context of routine veterinary anesthesia monitoring. Consequently, measurement of other parameters is necessary to ensure and maintain cardiovascular and pulmonary stability. These parameters can be broadly classified into one of three categories: monitors of circulation, monitors of oxygenation, and monitors of ventilation. Regardless of the techniques used, patient monitoring must involve regular repeated assessments and documentation of trends in order to make appropriate interpretations and management decisions. Provision of a suitably trained individual dedicated to anesthetic monitoring and case management is recommended for all patients, wherever possible.
Monitoring circulation
Circulatory efficiency would ideally be monitored using CO as a global indicator of blood flow in combination with measurement of organ-specific blood flow to ensure adequate perfusion to individual tissue beds. Unfortunately, this is not currently feasible in a clinical setting. There are, however, numerous parameters, both subjective and objective, that when used collectively constitute a reasonable approximation of circulatory efficiency in most patients.
Subjective methods
The assessment of mucous membrane color and capillary refill time (CRT) provides subjective information about peripheral vascular tone and perfusion. Pale mucous membranes (in the absence of anemia) suggest vasoconstriction and impaired perfusion while dark pink or red mucous membranes suggest vasodilation and increased perfusion. Similarly, prolonged CRT suggests vasoconstriction and impaired perfusion while rapid CRT suggests vasodilation. While subjective and qualitative in nature, these assessments should be part of the preanesthetic patient evaluation and should be performed in all patients at regular intervals (usually every 5 minutes) during anesthesia and the immediate recovery period.
Palpation of a peripheral arterial pulse provides subjective, qualitative information about the pulse pressure. Pulse pressure or “strength” is a function of the difference between systolic (SAP) and diastolic arterial pressures (DAP). Animals with large gradients between their systolic and diastolic pressures will have large pulse pressures and their peripheral pulses will feel “strong.” Patients with narrow gradients will have reduced pulse pressures and their peripheral pulses will feel “weak.” Note that this does not guarantee that patients with a strong peripheral pulse have adequate mean arterial pressure and peripheral perfusion. Thus, pulse palpation is not a substitute for quantitative ABP measurement.
Palpation of peripheral pulses for the purpose of assessing pulse rate (PR) and rhythm is, however, a clinically valuable skill and should be performed at frequent regular intervals in anesthetized animals for this purpose. Common sites for peripheral pulse palpation include the dorsal pedal, digital, and lingual arteries. In anesthetized cats, appreciation of peripheral pulses at these sites may be challenging and requires practice.
Objective methods
There are a number of different parameters that can be used to monitor circulatory status objectively. Some provide continuous data while others are performed intermittently and most require some sort of specialized equipment.
HR or PR
HR is one of the principle determinants of CO and maintenance of HR within a range appropriate for the individual patient and clinical scenario is necessary to ensure adequate circulatory stability. Normal resting HR in conscious dogs will vary among breeds but usually ranges from 70 to 120 beats per minute. In conscious cats, a true resting HR may be difficult to obtain in many clinical settings, but a range of 120–180 beats per minute is reasonable. Both bradycardia and tachycardia can have significant detrimental effects on CO and should be prevented and/or treated when they occur. Maintenance of HR within a “normal” range, however, in no way guarantees an adequate CO and other monitors are required to provide a more complete picture of circulatory status.
There are numerous techniques available for measurement of HR or PR. Some require the anesthetist to interact directly with the patient while some employ automated monitoring devices. Some techniques facilitate intermittent evaluations only while others provide continuous visual data and/or auditory signals. Regardless of the technique chosen, HR or PR should be monitored at least every 5 minutes in all anesthetized patients.
Thoracic auscultation of the heartbeat using a stethoscope is performed as part of the routine preanesthetic patient evaluation and may be used to document HR during anesthesia as well. This technique is simple and involves direct interaction with the patient on an intermittent basis. It is often a challenge for the anesthetist to access the patient’s thorax during various procedures, surgical and otherwise, so other alternatives must also be available.
Arterial pulse palpation is another technique that is useful in anesthetized patients. In dogs, common locations for pulse palpation include the femoral, dorsal pedal, digital, and lingual arteries. In cats, peripheral pulses are often more difficult to appreciate but can be located. As for auscultation, this technique involves direct patient interaction at frequent intervals and does not require any equipment. As there are a variety of arteries to choose from, at least one option is usually available in patients that are draped in for surgery. This technique is recommended for all anesthetized patients, even when other supplemental methods for HR assessment are used simultaneously.
The use of an esophageal stethoscope constitutes another low-cost method to evaluate HR. This technique involves esophageal placement of a probe that is attached to an earpiece and allows the anesthetist to appreciate heart sounds on an intermittent or semicontinuous basis. Alternatively, the probe may be attached to an electronic amplifier that projects a continuous audible signal. This method overcomes the inaccessibility issue for most patients and procedures and is recommended for routine use to supplement other automated monitoring techniques.
The Doppler system, though primarily utilized as a monitor of ABP, also provides an audible PR signal. This technique involves placement of a piezoelectric crystal over a peripheral artery (such as the palmar, dorsal pedal, or coccygeal) connected to an ultrasonic Doppler flow detector, which translates and amplifies the sound of peripheral blood flow. If the Doppler system is also to be used for blood pressure measurement, an occlusive cuff and sphygmomanometer must also be applied (see below). The flow detector can be left on for continuous audio signaling or turned on and off for intermittent evaluations. PR is not digitally displayed so the anesthetist must count the rate based on the audible signal.
The electrocardiogram (ECG), though primarily utilized as a monitor of cardiac rhythm, also provides a continuous digital display of HR. Many ECG monitors can be programmed to emit an audible beep with each beat. The accuracy of the HR displayed by the ECG monitor depends on the unit itself and patient factors such as size, lead placement, and ECG waveform morphology. With low amplitude QRS complexes and/or high rates (both of which are often seen in cats), some monitors may count inaccurately low rates or may not display an HR at all. Similarly, with large T wave amplitudes relative to the QRS complex, some monitors may double count and display an inaccurately high HR. Adjusting the settings on the ECG unit can often rectify these problems and produce a more reliable HR display. For these reasons; however, it is important to use supplemental techniques such as auscultation or pulse palpation to corroborate the HR displayed by an ECG unit.
The pulse oximeter, though primarily utilized as a monitor of oxygenation to measure percent hemoglobin saturation, also continuously displays a digital PR. Most pulse oximeters have an audible beep option corresponding to each detected pulse. The tongue is the most commonly used site for probe placement in anesthetized dogs and cats though other locations are possible. Some units also provide a plethysmographic waveform display, which, if consistently present, usually suggests that the displayed HR is reasonably accurate. Despite this, the use of other supplemental techniques such as auscultation or pulse palpation is recommended to corroborate the displayed PR on a pulse oximeter. It is important to remember that pulse oximetry is designed to noninvasively monitor oxygenation and is not suitable for assessing circulatory adequacy (beyond simply documenting the presence of a pulse and counting the rate).
Cardiac rhythm
Abnormalities of cardiac rhythm have the potential to significantly impact stroke volume (SV) and CO and, therefore, DO2. In some circumstances, documentation of a particular rhythm may signal an impending transition to a more serious, even life-threatening dysrhythmia. The challenge lies in not only recognizing that an abnormality is present but determining its potential significance. The only technique for quantitative evaluation of cardiac rhythm involves observation of cardiac electrical activity using the ECG. Figure 6.3 illustrates normal ECG waveform morphology. It is important to remember that cardiac electrical activity does not necessarily correlate with mechanical cardiac function. Documentation of normal cardiac rhythm in no way guarantees adequate CO and other methods of evaluating circulatory efficiency are required.
The most common approach to ECG monitoring in anesthetized dogs and cats involves placement of three surface electrodes, usually at the right forelimb (RA), left forelimb (L A), and left hind limb (LL) of the patient. This generates three bipolar limb leads for evaluation. Lead I is the voltage difference between the RA and LA electrodes, lead II the RA and LL electrodes, and lead III the LA and LL electrodes. Alternatively, a fourth electrode can also be placed on the right hind limb (RL) and this will generate three more leads referred to as augmented or unipolar limb leads (aVR, aVL, and aVF). Veterinary cardiologists may also place additional thoracic electrodes (rV2, V2, V4, and v10) to generate precordial leads that provide even more detailed diagnostic information regarding a particular dysrhythmia.
In most anesthetized patients, alligator clips with blunted teeth and relaxed spring mechanisms are used as electrodes. Alternatively, cutaneous adhesive pads may be placed after shaving an appropriate area of hair or by placing them on the foot pads. Depending on the procedure to be performed and patient positioning, electrode location may need to be adjusted. Application of conducting gel or 70% isopropyl alcohol where the electrodes contact the skin will improve signal transduction and may need to be repeated during long procedures.
Esophageal ECG electrodes are an alternative to standard surface electrodes in situations where skin placement may not be feasible (such as surgical procedures involving one or both proximal forelimbs) or where electrodes are likely to be disrupted during surgery. The electrode itself is placed in the esophagus and attached to a standard ECG cable.
With either surface or esophageal placement, the electrodes attach to a main cable that interfaces with a monitor. It may be a dedicated ECG monitor or, more likely, a multiparameter patient monitor capable of evaluating other parameters such as ABP, pulse oximetry, capnometry, temperature, and so on. The monitor will display a real-time tracing of cardiac electrical activity and the operator can select which of the three (or more) leads is displayed. The most commonly used lead for rhythm analysis in an anesthesia setting is lead II, but any lead demonstrating distinct P waves, QRS complexes, and T waves may be utilized. The speed of the tracing can be adjusted by the operator and is usually set at 25 or 50 mm/s. Other parameters such as the size of the complexes displayed can also be controlled. Some monitors have the capability to print ECG tracings out on paper and many newer models can interface with other types of monitoring equipment and have advanced data storage capabilities.
Several small handheld ECG units are also available that are completely electrode and cable free (such as the Vet Biolog™ II, VetLogix, LLC, Plymouth, MN). These are particularly useful for routine preanesthetic screening, for rapid diagnosis in emergency situations, or for anesthetic monitoring in any situation where use of a standard ECG monitor with electrodes and cables is cumbersome and/or not feasible.
ABP
ABP refers to the force exerted by circulating blood against arterial walls and is measured in millimeters of mercury (mm Hg). During each cardiac cycle, ABP varies between a maximum (SAP) and a minimum (DAP). Mean arterial blood pressure (MAP) is the average pressure over the entire cardiac cycle and, at resting HRs, can be estimated from SAP and DAP values as follows:
At high HRs, MAP more closely approximates the arithmetic mean of SAP and DAP as the shape of the pulse pressure narrows. Reported normal values for SAP and DAP in dogs and cats vary from publication to publication but tend to be in the range of 120–140 and 60–80 mm Hg, respectively. Reported values for MAP in conscious dogs and cats are more consistent, usually in the range of 90–100 mm Hg. The MAP is physiologically the most important of the pressures as it is considered to be the driving pressure for organ perfusion. Inadequate MAP over a sustained period of time may result in end-organ ischemia and must be avoided. Hemodynamically, MAP is determined by three factors: CO, systemic vascular resistance (SVR), and central venous pressure (CVP) as illustrated in Figure 6.1 and shown below:
CO is a primary determinant of DO2 while SVR is the resistance to blood flow within the systemic vasculature. It is primarily a function of vasomotor tone in small arterioles. CVP is the average pressure in the venous compartment and is a major determinant of right ventricular filling pressure and preload and therefore SV. Because CVP is usually at or near zero, the above relationship is often simplified as follows:
An appreciation of this relationship is crucial in understanding how ABP measurement fits into the larger circulatory picture. When monitoring ABP in anesthetized patients in the absence of CO data, as is usually the case, consideration must be given to the patient’s vasomotor status. Inadequate MAP is referred to as hypotension and may be the result of inadequate HR or SV causing reduced CO, vasodilation, or a combination of both. High MAP is referred to as hypertension and may be the result of increased blood volume and CO according to the Frank–Starling relationship, or vasoconstriction. It is important to recognize that observation of a normal MAP does not imply that CO is adequate. Based on the above relationship, a patient may have very poor CO, but be normotensive if concurrently vasoconstricted. Thus, the clinician must make a judgment regarding the patient’s myocardial function and vasomotor status in order to fully interpret the significance of a particular ABP measurement.
Clinical measurement of ABP may involve noninvasive approaches such as the oscillometric or Doppler techniques, or the invasive technique involving placement of an arterial catheter. All noninvasive techniques provide estimates of ABP and may not be absolutely accurate in all situations. In most cases, however, noninvasive methods effectively reflect trends in ABP and are recommended for routine use during anesthetic monitoring in all dogs and cats. Invasive ABP measurement is the most accurate approach but is not without limitations or complications. Each of these options is discussed below.
The oscillometric method for ABP determination is an automated, noninvasive (indirect) system that involves placement of a pneumatic occlusion cuff around a peripheral limb or the base of the tail. The cuff is automatically inflated to a suprasystolic pressure and then air is gradually released until characteristic arterial oscillations are detected by an electronic sensor. The computer interprets these oscillations and displays measured values for SAP, MAP, and DAP. Oscillometric monitors also display a PR as well. They can be programmed to automatically cycle at regular intervals, which is convenient when monitoring anesthetized patients. The width of the occlusion cuff is critical for accurate measurements and should be approximately 40% the circumference of the extremity around which it is placed. Cuffs that are too small will overestimate ABP while cuffs that are too large will underestimate it.
Traditionally, oscillometric ABP monitors available to veterinarians were designed for use in adult human patients, and the algorithms where not always able to detect oscillations in the arteries of very small canine and feline patients. In addition, the HR range was not appropriate for many small veterinary patients. Consequently, many such monitors were inaccurate or simply not able to provide measurements in small patients. As ABP monitoring has become increasingly common in veterinary medicine, a number of veterinary-specific oscillometric monitors have been marketed (such as the Cardell® [MidmarkAnimal Health, Versailles, OH], PetMAP™ [Ramsey Medical, Inc. Tampa, FL], and BP-AccuGard™ [Vmed Technology, Mill Creek, WA] monitors). These monitors appear to function more reliably in a wider range of veterinary patients, though inaccuracy at extremes of ABP remains an issue. Oscillometric ABP monitors are available as stand-alone units or, as veterinary anesthetic monitoring becomes increasingly sophisticated, as part of multiparameter units that also have ECG, pulse oximetry, capnography, and temperature capabilities.
The Doppler system for ABP measurement is a noninvasive (indirect) system that involves placement of a piezoelectric crystal over a peripheral artery such as the digital, dorsal pedal, or coccygeal. Blood flow to and from the crystal reflects sound waves causing a change in frequency that is detected, translated, and amplified to a speaker. In order to use the Doppler system to determine ABP, an appropriately sized occlusive cuff (40% the circumference of the extremity) is then placed proximal to the probe location and connected to a sphygmomanometer. The cuff is inflated until the audible blood flow signal is lost and then incrementally deflated until the return of flow is recognized. The pressure displayed on the sphygmomanometer at the point when this occurs corresponds to the SAP.
Doppler systems (such as the Parks Medical Model 811 [Parks Medical Electronics, Las Vegas, NV] and VMed Vet-Dop™ [Vmed Technology] monitors) are not automated, so manual SAP determination by the anesthetist is required at intervals. It is not possible to determine MAP or DAP values with this system and its accuracy for determination of SAP is influenced by a number of factors. In anesthetized small patients (cats and small dogs), Doppler pressure readings tend to underestimate SAP. Some studies involving cats have suggested that SAP values are undervalued by approximately 15 mm Hg and that the Doppler measurement may actually be a more accurate reflection of MAP in these patients. Consequently, like any noninvasive ABP monitor, the Doppler system is best used as an indicator of trends when used for anesthetic monitoring.
Invasive or direct ABP measurement provides an accurate, quantitative, continuous assessment of SAP, MAP, and DAP, and also provides a graphical representation of the arterial pulse waveform as illustrated in Figure 6.4. This technique requires placement of an arterial catheter, usually in a peripheral artery such as the dorsal pedal, carpal or coccygeal, and thus is more technically challenging than noninvasive approaches. The catheter is then connected to saline-filled, semirigid arterial pressure tubing, which is in turn connected to an electronic resistance-type pressure transducer. The transducer should be placed at the level of the patient’s right atrium to obtain accurate ABP measurements. Modern monitors typically have an offset pressure that allows the transducer to be moved to any convenient level and the monitor can compensate for any vertical difference between transducer and patient. The transducer connects to a cable that interfaces with a multiparameter patient monitor that displays the arterial pressure waveform in real time along with digital values for SAP, MAP, DAP, and PR. Recently, a number of veterinary specific multiparameter patient monitors have been marketed with direct pressure capabilities.
While direct ABP measurement is considered the gold standard, it is not without potential sources for error and complications. The waveform can become dampened if the catheter tip is up against the arterial wall, if a clot forms at the catheter tip, if air bubbles are present within the catheter or tubing, or if the catheter or tubing becomes kinked. This will result in a flattened arterial waveform, lowered reported values for SAP, and higher reported values for DAP. The use of compliant tubing instead of semirigid arterial monitoring tubing will also negatively impact the fidelity of the system. Also, because this technique requires arterial catheterization, potential complications such as hematoma formation, infection, thrombosis, or necrosis of tissues distal to the catheter site are possible (though not common). Heparinization of the line to maintain its patency is required in most situations and this may be a concern in very small patients. Direct ABP measurement is also associated with an increased cost compared to noninvasive methods as it requires a number of consumable products (catheter, tubing, and transducer) in addition to the upfront cost associated with purchase of the monitor. In patients with preexisting or potential circulatory instability, however, this method is superior for monitoring during anesthesia and in critical care settings.
CVP
CVP is an estimate of mean right atrial pressure and reflects the balance between CO and venous return. Primary factors influencing CVP include blood volume, venous tone, cardiac contractility, HR, changes in intrathoracic or intrapericardial pressures, and body position. In dogs and cats, normal CVP ranges from 0 to 10 cm H2O. Decreases in CVP generally indicate absolute or relative hypovolemia, while increases indicate hypervolemia or myocardial depression and/or heart failure.
CVP measurement requires placement of a catheter into the jugular vein extending to the level of the thoracic vena cava or, preferably, the right atrium. In cats, caudal vena cava pressures are a reasonable surrogate for CVP when a jugular catheter cannot be placed. The catheter is flushed with saline and connected to a commercially available manometer using saline-filled tubing attached to a three-way stopcock. A saline-filled fluid administration line is connected to the stopcock port immediately across from the catheter, and the manometer is attached to the remaining port. With the stopcock closed to the patient the graduated cylinder is filled with saline solution. To obtain an accurate measurement the three-way stopcock is positioned just below the base of the heart. In laterally recumbent animals this corresponds to the sternum, and in dorsally recumbent animals to the point of the shoulder. The stopcock is then closed to the fluid administration set and the resulting CVP measurement is read off of the gradations on the manometer in cm H2O.
Alternatively, the catheter can be connected to a pressure transducer and interfaced with a multiparameter patient monitor similar to direct ABP measurement. Values for CVP and the characteristic CVP waveform will be digitally displayed. If using a standard pressure channel, most monitors will report CVP in millimeters of mercury, which must be converted to centimeters of water for interpretation (1 mm Hg = 1.36 cm H2O). While CVP measurement is not indicated for routine anesthetic monitoring in healthy dogs and cats it does provide useful information in patients requiring aggressive volume resuscitation, especially in the face of concurrent myocardial dysfunction.
Cardiac output
CO is a principle determinant of DO2 and an important indicator of global circulatory adequacy. Unfortunately, truly definitive conclusions about CO cannot be made on the basis of ABP and/or CVP measurements. Because CO is a flow parameter as opposed to a pressure parameter it is logistically more challenging to measure and, traditionally, has required invasive techniques such as cardiac catheterization. Consequently, normal values for CO in conscious dogs and cats based on large numbers of animals and indexed to body surface area are not well established but are probably in the range of 4.0L/min/m2 (or approximately 140–160mL/min/kg).
Though measurement of CO has been impractical in most clinical veterinary settings, including anesthetic monitoring, this may change in the future. As the biomedical industry strives to find new noninvasive or minimally invasive methods of CO measurement for use in humans, veterinary patients may indirectly benefit. Note that the following discussion covers a few selected techniques only and is in no way meant to be an exhaustive review on the subject of CO measurement.
The traditional approach to clinical CO measurement is called the thermodilution technique. It is considered invasive as it involves placement of a balloon-tipped pulmonary arterial (Swan–Ganz) catheter. A small, known volume of fluid at a known temperature is injected into the catheter’s proximal pulmonary arterial port and the blood temperature is subsequently measured at a thermistor located a known distance away along the catheter. A computer generates and interprets a temperature-time (thermodilution) curve and calculates CO as being inversely proportional to the area under this curve. Use of this method in human patients is in decline as more user-friendly, less invasive options with fewer potential complications are developed.
A newer system, also based on indicator dilution, avoids the need for cardiac catheterization. It requires peripheral venous and arterial catheterization only and incorporates the lithium dilution technique (LiDCO™, LidCO Ltd., Sawston, Cambridge UK). A small dose of lithium chloride is injected into the venous line and the resulting arterial lithium concentration-time curve is recorded by withdrawing blood past a lithium sensor attached to the arterial line. The computer than calculates and reports a value for CO.
A more recent technology has combined the LiDCO system with a variation on pulse contour analysis. Traditional pulse contour analysis systems attempted to measure SV on a beat-to-beat basis by analyzing the arterial pulse pressure waveform. There were a number of drawbacks associated with this approach but a new system, called arterial pulse power analysis (PulseCO™, LidCO Ltd.), uses an algorithm that overcomes many of these obstacles. The combination of these two technologies (LiDCO and PulseCO) into the LiDCOplus Hemodynamic Monitor (LidCO Ltd.) provides real-time, continuous assessment of CO for use in patients and requires peripheral venous and arterial access only.
An alternative technique is based on the Fick principle using partial rebreathing of CO2. This monitor (NICO®, Philips Respironics, Murrysville, PA) estimates CO based on changes in end-tidal CO2 (ETCO2) concentration caused by brief intermittent periods of rebreathing through a specific disposable loop. The monitor consists of a carbon dioxide sensor (infrared light absorption), an airflow sensor (differential pressure pneumotachometer), and a pulse oximeter. The partial rebreathing reduces CO2 elimination and increases ETCO2. Patients must be mechanically ventilated and arterial blood sampling is required so that arterial oxygen tension (PaO2) can be entered into the monitor for shunt estimation, which may otherwise skew CO results.
Transesophageal echocardiography (TEE) uses Doppler ultrasonography to measure blood velocity in the descending aorta using a transducer located at the tip of a flexible esophageal probe. The resultant velocity-time integral is multiplied by the measured aortic cross-sectional area to get blood flow, which is then multiplied by HR. Estimation of CO by this method assumes a fixed ratio of blood flow between the descending aorta and the left ventricular outflow tract since only descending aortic flow is actually measured. This technique requires training in obtaining appropriate echocardiographic images and making accurate aortic measurements.
More recently, a completely noninvasive continuous-wave Doppler ultrasound technology specifically designed to assess CO has been introduced (USCOM, USCOM Pty Ltd, Coffs Harbour, NSW, Australia). The USCOM monitor transcutaneously measures the Doppler flow profile velocity time integral but uses anthropometry to calculate aortic and pulmonary valve diameters so both right- and left-sided flows can be measured. This technique is technically simple to perform and does not require advanced training in ultrasonography. While it is unlikely that the anthropometric scaling used by the USCOM for human patients will translate well across all breeds of canine patients, which vary widely in stature, it is likely that some form of noninvasive CO assessment will be possible in veterinary medicine in the near future.
Oxygenating efficiency would ideally be monitored by measurement of total CaO2 as it incorporates the fraction of oxygen bound to hemoglobin as well as the fraction dissolved in plasma. Though the key contributors to CaO2 (i.e., SaO2, [Hb], and PaO2) can be accurately measured, practically, it is not always necessary to evaluate all of them in the context of anesthetic monitoring. Because PaO2 and SaO2 are related to each other according to the oxyhemoglobin dissociation curve, measurement or estimation of one parameter offers insight into the other. Note that the position of the dissociation curve is, however, affected by a number of other factors. Increases in hydrogen ion concentration (i.e., decreased pH), pCO2, temperature, and 2,3-diphosphosglycerate (2,3-DPG) levels all shift the curve to the right. Figure 6.5 illustrates the canine oxyhemoglobin dissociation curve under normal conditions (i.e., with a P50 value of approximately 28 30 mm Hg). The feline curve under normal conditions appears similar but has a P50 value of approximately 32–37 mm Hg and thus is shifted rightward compared to dogs and humans.
Figure 6.5. Canine oxyhemoglobin dissociation curve under normal conditions (P50 approximately 28–30 mm Hg).

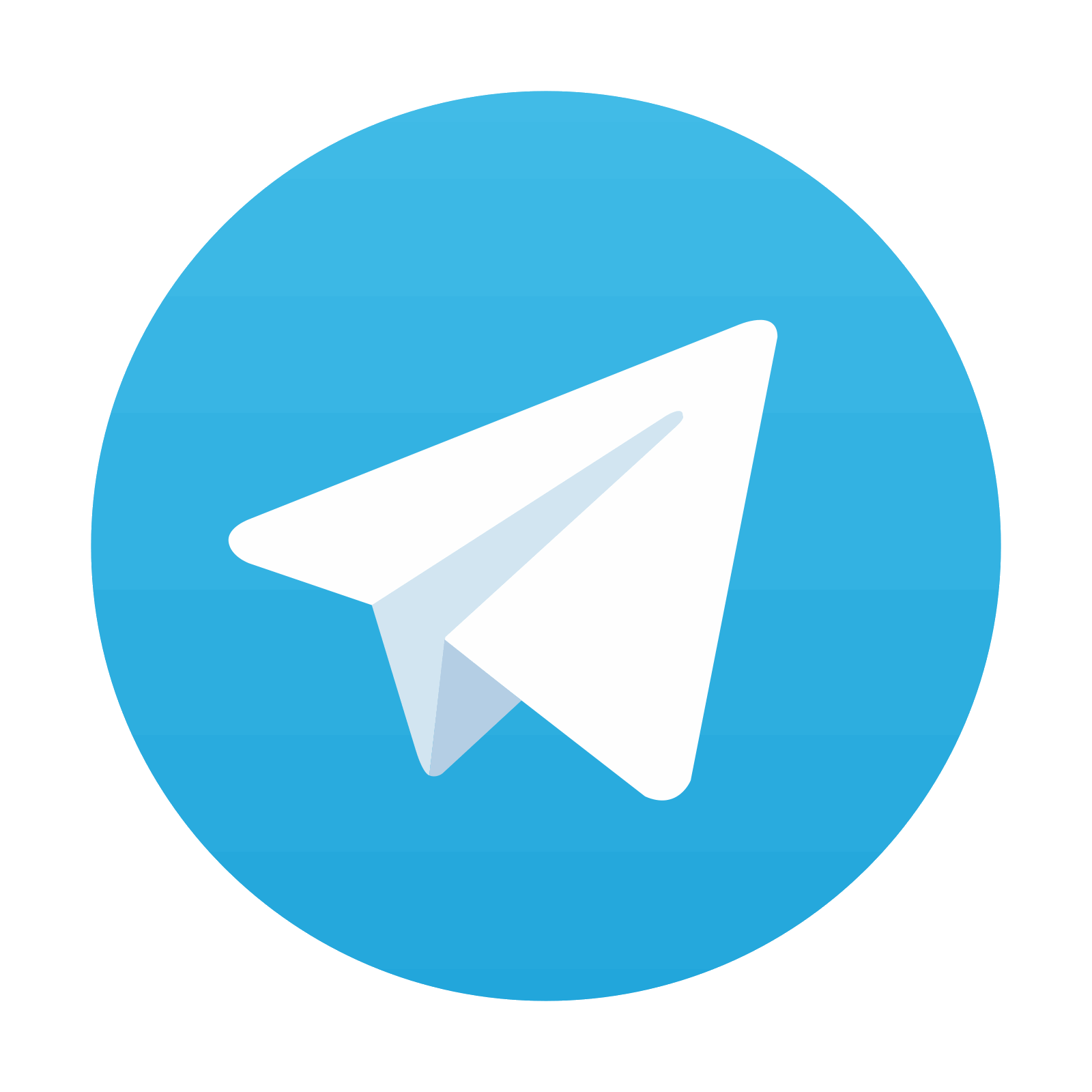
Stay updated, free articles. Join our Telegram channel
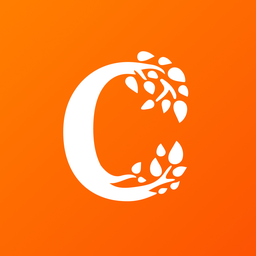
Full access? Get Clinical Tree
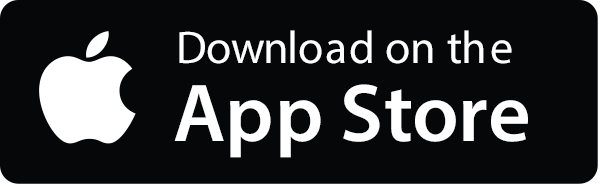
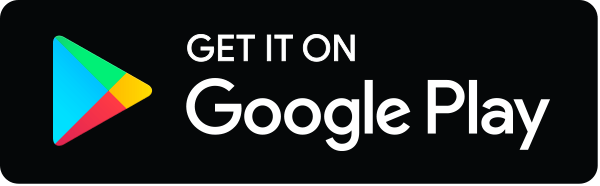