Introduction
The prevention and control of pain are central to the practice of veterinary medicine. It is essential that clinicians have an understanding of the physiological processes leading to the perception of pain and the responses of patients to this process. Ultimately, anesthetic patient management is the control of pain and maintenance of homeostasis in the face of noxious stimuli. The perioperative analgesic protocol has an impact on patient well-being that often extends far beyond the immediate anesthetic period. Appropriate pain management is not only integral to an anesthetic plan; it is a fundamental component of good medical practice.
Pain is an unpleasant sensory and emotional experience (perception) associated with actual or potential tissue damage or is described in terms of such damage. The inability to communicate in no way negates the possibility that an individual is experiencing pain and is in need of appropriate pain-relieving treatment.
Nociceptive pain arises from the activation of a discrete set of receptors and neural pathways by noxious stimuli that are actually or potentially damaging to tissues. Pain is a conscious awareness of acute or chronic nociceptive stimulation occurring in varying degrees of severity resulting from injury and disease, or abnormal neural processing associated with emotional distress as evidenced by biologic or behavioral changes or both. Pain elicits protective motor actions, results in learned avoidance, and may modify species-specific traits of behavior, including social behavior. Acute pain is the result of a traumatic, surgical, or infectious event that begins abruptly and is relatively brief. It is generally alleviated by analgesic drugs. Chronic pain is pain that persists beyond the usual course of an acute disease or beyond a reasonable time for an injury to heal, or that is associated with a chronic pathological process or neurological dysfunction that persists or recurs for months or years (e.g., osteoarthritis). Chronic pain is seldom permanently alleviated by analgesics, but may respond to a combination of analgesics, tranquilizers or psychotropic drugs, physical therapy, environmental manipulation, and behavioral conditioning. Acute pain is a symptom of disease, whereas chronic pain, in and of itself, is a disease of altered neuroprocessing. Acute pain has a biologic function in that it serves as a warning that something is wrong and leads to protective behavioral changes. Chronic pain does not serve a biologic function and imposes severe detrimental stresses. Because pain is a perception, it is always subjective.
In people, pain experience has three dimensions—sensory-discriminative, motivational affective, and cognitive-evaluative—that are subserved by physiologically distinct systems. The sensory-discriminative dimension provides information on the onset, location, intensity, type, and duration of the pain-inducing stimulus. This aspect is subserved primarily by the lateral ascending nociceptive tracts, thalamus, and somatosensory cortex. The motivational-affective dimension disturbs the feeling of well-being of the individual, resulting in the unpleasant experience of pain and suffering, and triggers the organism into action. This dimension is closely linked to the autonomic nervous system, and cardiovascular, respiratory, and gastrointestinal responses are associated with it (although these can also occur reflexly). This dimension is subserved by the medial ascending nociceptive tracts and their input into the limbic system. The cognitive-evaluative dimension encompasses the effects of prior experience, social and cultural values, anxiety, attention, and conditioning. These activities are largely caused by cortical activity, although cortical activation is dependent on reticular activity. The cognitive-evaluative dimension of the pain experience in lower mammals may be the only one that differs significantly from that in people.
To discuss pain physiology and its management requires a review of the definitions commonly used to describe this perception.
Agology The science and study of pain phenomena.
Allodynia Pain caused by a stimulus that does not normally provoke pain.
Analgesia The absence of pain in the presence of stimuli that would normally be painful. Analgesics Drugs that produce analgesia.
Anesthesia The absence of all sensory modalities.
Anesthetics Drugs that induce regional anesthesia (i.e., in one part of the body) or general anesthesia (i.e., unconsciousness).
Cancer pain Pain that is caused by primary tumor growth, metastatic disease, or the toxic effects of chemotherapy and radiation, such as neuropathies caused by neurotoxic antineoplastic drugs.
Causalgia A syndrome of prolonged burning pain, allodynia, and hyperpathia after a traumatic nerve lesion, often combined with vasomotor and sudomotor (sweating) dysfunction and later trophic changes.
Central pain Pain associated with a lesion or altered neurophysiology of the central nervous system (CNS).
Chronic pain Pain that persists for longer than the expected time frame for healing or pain associated with progressive nonmalignant disease (such as osteoarthritis).
Deafferentation pain Pain caused by loss of sensory input into the CNS, as occurs with avulsion of the brachial plexus or other types of peripheral nerve lesions, or caused by pathology of the CNS.
Dermatome The sensory segmental supply to skin and subcutaneous tissue.
Distress The external expression through emotion or behavior (i.e., fear, anxiety, hyperactivity, or aggression) of suffering.
Dysesthesia An unpleasant abnormal sensation, whether spontaneous or evoked.
Hyperalgesia An increased response to a stimulation that is normally painful.
Hyperesthesia An increased sensitivity to stimulation, excluding special senses.
Hypoalgesia A diminished sensitivity to noxious stimulation.
Hypoesthesia A diminished sensitivity to stimulation, excluding special senses.
Inflammatory pain Spontaneous pain and hypersensitivity to pain in response to tissue damage and inflammation.
Neuralgia Pain in the distribution pathway of a nerve or nerves.
Neuritis Inflammation of a nerve or nerves.
Neuropathic pain Spontaneous pain and hypersensitivity to pain in association with damage to or a lesion of the nervous system.
Neuropathy A disturbance of function or a pathological change in a nerve.
Nociception The reception, conduction, and central nervous processing of nerve signals generated by the stimulation of nociceptors. It is the physiological process that, when carried to completion, results in the conscious perception of pain.
Nociceptive pain Transient pain in response to noxious stimuli.
Nociceptor A receptor preferentially sensitive to a noxious stimulus or to a stimulus that would become noxious if prolonged.
Nociceptor threshold The minimum strength of stimulus that will cause a nociceptor to generate a nerve impulse.
Noxious stimulus One that is actually or potentially damaging to body tissue. It is one of intensity and quality that are adequate to trigger nociceptive reactions in an animal, including pain in people.
Pain (detection) threshold The least experience of pain that an individual can recognize. The point at which an individual just begins to feel pain when a noxious stimulus is being applied in an ascending trial or the point at which pain disappears in a descending trial. The pain-detection threshold is relatively constant among individuals and species. In most cases, it is higher than the nociceptor threshold.
Pain tolerance The greatest level of pain that an individual will tolerate. Pain tolerance varies considerably among individuals, both human and animal. It is influenced greatly by the individual’s prior experience, environment, stress, and drugs.
Pain-tolerance range The arithmetic difference between the pain-detection threshold and the pain-tolerance threshold.
Paresthesia An abnormal sensation, whether spontaneous or evoked. Paresthesias are not painful (as opposed to dysesthesias).
Radiculalgia Pain along the distribution of one or more sensory nerve roots.
Radiculitis Inflammation of one or more nerve roots.
Radiculopathy A disturbance of function or pathological change in one or more nerve roots.
Reactions A combination of reflexes designed to produce widespread movement in relation to the application of a stimulus. Reactions are mass reflexes not under voluntary control and therefore do not involve the cerebral cortex.
Reflexes Involuntary, purposeful, and orderly responses to a stimulus. The anatomical basis for the reflex arc consists of a receptor, a primary afferent nerve fiber associated with the receptor, a region of integration in the spinal cord or brain stem (synapses), and a lower motor neuron leading to an effector organ such as skeletal muscles (somatic reflexes), smooth muscles, or glands (visceral reflexes).
Responses Willful movement of the body or parts of the body. A response cannot occur without involvement of the somatosensory cerebral cortex. A decerebrate animal can give a reaction but not a response. Reflexes and reactions may or may not be perception linked (i.e., the stimulus perceived as painful). Because responses require a functioning somatosensory cortex, the initiating stimulus must first be perceived.
Somatic Usually used to describe input from body tissues other than viscera.
Suffering An unpleasant emotional state that is internalized and not expressed outwardly. It is described as an undesirable mental state or as an unpleasant emotion that people or animals would normally prefer to avoid. Suffering can refer to a wide range of intense and unpleasant subjective states, such as fear and frustration. It can be of either physical or psychological origin. Suffering can be provoked by pain or by pain-free nontissue-damaging external stimuli such as denial of the fulfillment of an animal’s natural instincts or needs, such as maternal deprivation, social contacts, and so on.
Neuroanatomy of nociceptive pathways
Nociceptors and stimuli
Nociception is the reception of signals from activation of nociceptors, which are receptors that detect tissue-damaging (noxious) stimuli. Pain implies that noxious stimuli have been perceived at the cortical level. Activating stimuli for nociceptors can include mechanical, thermal, or chemical stimuli. Some nociceptors respond only to one of these modalities, whereas others are sensitive to a variety of them (polymodal nociceptors). Nociceptors are naked (nonencapsulated) nerve endings, widely distributed in skin and deep tissues. These represent the peripheral termini of nociceptive primary afferent neurons that possess lightly or unmyelinated, small-diameter axons. Activation of fastconducting (5–30m/s), A-δ fibers are associated with sharp, pricking pain (as reported by humans). Slow-conducting (0.5–2.0m/s), unmyelinated C fibers are associated with a slower, burning type of pain. Both types of nociceptive fibers innervate the skin (superficial pain) and deep somatic or visceral structures (deep pain). The distinction between superficial and deep pain is not just an arbitrary one based on “outside” and “inside.” Each is associated with an anatomically and functionally segregated central pain pathway; they are differentially susceptible to injury, and they are examined separately in a neurological exam.
Pain research has revealed the presence of a particular functional type of nociceptor referred to as a silent nociceptor. The high threshold of this nerve ending ensures that under normal circumstances it is relatively insensitive to any stimuli. Following release of tissue-inflammatory mediators, however, the threshold is markedly reduced, and these previously silent nociceptors can be activated by a variety of thermal and mechanical stimuli. The presence of silent nociceptors is one mechanism by which inflammation produces primary hyperalgesia.
Divergence in nociceptive pathways
In addition to the connections of ascending nociceptive pathways with somatosensory cortex for conscious perception, nociceptive pathways exhibit variable degrees of connectivity with a number of subcortical regions of the brain, and through these connections elicit a variety of nonconscious responses.
A behaviorally important aspect of nociception (and other sensory modalities) is the degree to which it affects mental alertness. This relationship between sensation and consciousness is orchestrated in the reticular formation (RF), a loose aggregate of nuclei in the central core of the brain stem, extending from the diencephalon through the medulla oblongata. Functions of the RF include regulation of heart and respiratory rates, selective attention to stimuli, and maintenance of consciousness and cortical alertness. The RF is critical to the regulation of the level of consciousness through its rostral projections to the diencephalon, which, in turn, diffusely excites the cerebral cortex. The RF receives input from all afferent pathways, although the degree to which these connections are made is variable, depending on the pathway. Stimuli—but most especially noxious stimuli—increase alertness and autonomic functions, such as heart and respiratory rates.
Nociceptive information is simultaneously directed to the hypothalamus, which is the brain’s coordinator of elaborate autonomic responses and the primary integrator of physiological and emotional responses. Input from nociceptive pathways to the hypothalamus produces activity in the sympathetic nervous system and the pituitary gland and thus increases circulating epinephrine/norepinephrine and glucocorticoids. The catabolic and other endocrinologic manifestations of this activation can have negative effects on health.
Both the hypothalamus and the RF have projections to other parts of the limbic system, a group of cortical and subcortical regions that produce the behavioral, cognitive, and physiological changes that people describe as emotions.
Nociceptive pathways send collateral projections to the mesencephalon (midbrain). One set of nuclear targets in the midbrain consist of motor neurons that coordinate orienting movements of the head and eyes toward the noxious stimulus (the visual grasp reflex). Other neurons that form the periaqueductal gray matter (PAG) of the midbrain activate important descending pain modulatory systems.
Ascending spinal pathways
Multiple nociceptive pathways that have been described in the spinal cord of domestic animals are present in all funiculi of the cord and with a confusing degree of overlap in their functions. None of these pathways are exclusive for transmission of nociception (all have fibers conducting tactile information). For clinical purposes, only two need be understood fully: the spinocervicothalamic and spinoreticular tracts.
The spinocervicothalamic tract is concerned with the transmission of superficial pain and tactile sensations and is regarded as the primary conscious pain pathway in carnivores. The primary afferents of this pathway synapse in the dorsal horn, from which secondary afferents then mediate local reflexes and project craniad in an ipsilateral tract in the dorsal part of the lateral funiculus. The axons in this tract ascend to spinal cord segments C1 and C2, where they synapse in the lateral cervical nucleus. The fibers arising from this nucleus will then decussate and project through the brain stem to the thalamus. Some collaterals of the ascending fibers will terminate in the RF. From the thalamus, fibers project to the somatosensory cortex.
The sensations transmitted by the spinocervicothalamic tract are touch and superficial pain. This pathway is discriminative in that the location of the painful stimulus can be precisely determined by the animal, which is a quality linked to the high degree of somatotopy exhibited by this pathway. Clinically, the function of the spinocervicothalamic tract is tested by lightly pinching the skin with fingers or a mosquito hemostat. This stimulus is applied lightly and briefly so as to activate the spinocervicothalamic pathway preferentially.
The spinoreticular tract is primarily concerned with transmission of deep-pain and visceral sensations. The primary afferents of this pathway enter the cord and immediately diverge to send collaterals several segments rostral and caudal to the segment of entry. This spreading of information across several spinal cord segments enables these afferents to participate in intersegmental reflexes (various manifestations of withdrawal and postural reflexes in response to painful stimuli). Second-order neurons are found in the dorsal horn. Axons of projection neurons in this system are present diffusely in the lateral and ventral funiculi. These projections are bilateral; decussation of axons in this system occurs diffusely throughout the long axis of the spinal cord.
Most ascending projections of the spinoreticular tract that reach the brain stem do not project directly to the thalamus; rather, they terminate in the RF. Therefore most deep pain that is consciously perceived arrives at the cortex via diffuse reticular projections to the thalamus. Activation of this pathway increases arousal and activates the limbic system, a connection that in humans is associated with emotional responses to pain.
Visceral pain is particularly poorly localized. Visceral afferent fibers travel in sympathetic nerves, have large overlapping receptor fields, and respond primarily to stretch, ischemia, dilation, or spasm (direct trauma to viscera—including surgical trauma—is a surprisingly ineffective stimulus for nociceptors). Pain of visceral origin tends to be dull, aching, or burning. Primary afferents from viscera follow autonomic nerves (e.g., the vagus and sympathetic nerves) to the CNS. The deep nociceptive pathway is tested in the neurological examination by application of a hemostat across the base of a toenail (taking care to exclude skin), a manipulation that stimulates nociceptors in the periosteum of the third phalanx.
Trigeminal system
For the head, nociception and tactile information are transmitted by the trigeminal system. Cell bodies of primary afferent fibers reside within the trigeminal (semilunar) ganglion. Their central processes enter the pons with the trigeminal nerve and course caudal along the lateral surface of the medulla. A nuclear column lies medial to the spinal tract throughout its length. At its rostral extent in the pons, this group of cell bodies comprises the pontine sensory nucleus. More caudally, the column is known as the spinal nucleus of V. The pontine and spinal nuclei of V contain somata of the second-order neurons in this system. There is a rostral-to-caudal segregation of function in these nuclei; the pontine nucleus is primarily concerned with discriminative tactile and proprioceptive stimuli, and the rostral part of the spinal nucleus of V sends somatosensory information to the cerebellum. The majority of neurons in the spinal nucleus of V, however, are concerned with nociception. Many of these will project to motor nuclei of cranial nerves to participate in reflex arcs (e.g., corneal and palpebral reflexes), and many more will project to the RF to affect autonomic responses and increase arousal. Fibers for conscious perception, however, cross the midline of the medulla diffusely and join the contralateral quintothalamic tract, adjacent to the medial lemniscus. The quintothalamic (also known as the trigeminal lemniscus) tract projects to the thalamus, and, from there, nociceptive information reaches the somatosensory cortex via the internal capsule.
There is a tendency to conceive of somatosensory pathways as electrical circuits that respond to stimuli in predictable ways and that consistently produce a sensory perception that is a faithful recording of the stimulus in the periphery. This is a useful model but it grossly oversimplifies the actual condition, wherein activity in the CNS can modulate somatosensory processing. This ability to alter activity in sensory systems is especially well developed in nociceptive pathways. The ability of a given stimulus to produce a perception of pain is a highly labile property and can be modified in the periphery, in the spinal cord, in the brain stem, and in higher centers.
Modulation in the periphery (nociceptors)
Nociceptor threshold is not a constant. As was described above, the presence of so-called silent nociceptors is one example of how conditions in the cellular environment of the naked nerve ending can change the sensitivity of the receptor to stimulus. The high threshold of silent nociceptors ensures that, under normal circumstances, they are relatively insensitive to any stimuli, but, upon exposure to inflammatory mediators, this threshold is markedly reduced, and previously silent nociceptors can be activated.
Similarly, many inflammatory mediators (e.g., prostaglandins [PGs] and leukotrienes), collectively referred to as nociceptor sensitizers, will lower the threshold of other populations of nociceptors. Thus, in damaged or inflamed tissue, stimuli that would normally be subthreshold may produce activity in nociceptive afferents. Likewise, certain inflammatory mediators (e.g., bradykinin and serotonin) or substances released by damaged cells (e.g., potassium ions and adenosine triphosphate) directly stimulate nociceptors and can thus be considered nociceptor activators. Interestingly, stimulated free-nerve endings can release substances directly into the surrounding tissues. Notable among these is substance P. Substance P (which is also an important neurotransmitter in central nociceptive pathways) dilates blood vessels and degranulates mast cells (neurogenic edema), both of which contribute to inflammation and increased sensitization of local nociceptors. All of these events contribute to the development of primary hyperalgesia (resulting from the increased responsiveness of nociceptors to noxious stimuli) and a related phenomenon, allodynia (wherein normally nonnoxious stimuli, such as those that elicit a touch sensation, become capable of activating nociceptors).
Modulation in the dorsal horn
Considerable processing of nociceptive information occurs in the dorsal horn, although precisely what happens there is debated. One of the fundamental concepts of dorsal horn processing is that one population of second-order neurons is dedicated to nociception (nociceptive-specific cells) and an additional, smaller group receives input from primary afferents conducting both noxious and nonnoxious tactile information. It is suspected that the nociceptive-specific neurons are primarily involved in discriminative nociception (i.e., localization). The second group, referred to as wide-dynamic-range or WDR neurons, responds both to noxious and nonnoxious stimuli, and is likely to be recruited in pathways that exhibit less somatotopy (i.e., are less discriminative). The WDR neurons appear to be relatively insensitive to tactile information, discharging at one rate in response to innocuous touch stimuli, while responding more vigorously (at a greater frequency) to noxious stimulation. These neurons also receive information from both somatic and visceral structures, which is a feature believed to underlie the phenomenon of referred pain. In referred pain, noxious stimuli originating in viscera are perceived as originating instead from a somatic region (body wall or skin). This perception is thought to result from the fact that information from that region of viscera converges on WDR neurons and pathways that also convey information from somatic structures.
The WDR neurons are probably the cells most important in the expression of spinal facilitation of pain, or windup (Figure 3.1). Windup occurs with rapid, continuous firing of primary nociceptive afferents, probably most especially small-diameter, unmyelinated fibers (C fibers). The high-frequency volley of action potentials (APs) in the primary afferent terminal stimulates the release of increased amounts of glutamate and is also associated with the release of substance P and brain-derived neurotrophic factor (BDNF). The increased exposure to glutamate in the synaptic cleft activates N-methyl-d-aspartate (NMDA) receptors (inactive except under conditions of persistent membrane depolarization) on the postsynaptic membrane. This particular variety of glutamate receptor is unique in that it exhibits a calcium conductance; its activation is therefore associated with the influx of calcium ions onto the postsynaptic neuron, leading to a series of intracellular cascades that ultimately results in the upregulation of receptors. Substance P and BDNF are neuromodulatory neurotransmitters that bind with G protein-coupled receptors. These, too, activate intracellular signaling cascades that increase the membrane’s sensitivity to subsequent stimulation.
Figure 3.1. Processes involved in the spinal facilitation of pain or windup. AMPA, α-amino3-hydroxy-5-methyl-4-isoxazolepropionic acid; AP, action potential; BDNF, brain-derived neurotrophic factor; NMDA, N-methyl-d-aspartate.
Source: Hellyer P.W., Robertson S.A., Fails A.D. 2007. Pain and its management. In: Lumb and Jones’ Veterinary Anesthesia and Analgesia, 4th ed. W.J. Tranquilli, J.C. Thurmon, and K.A. Grimm, eds. Ames, IA: Blackwell Publishing, p. 37.

The net effect of these events is that high-frequency APs in the primary afferent neuron “train“ the second-order neurons to respond more vigorously to subsequent stimulation. This change can last from hours to days (and longer) after the causative event ends. Effectively, then, prolonged noxious stimuli produce greater sensitivity to subsequent stimuli. What is especially significant about this phenomenon is that general anesthesia does not prevent windup, as it does not prevent generation of APs in the primary afferents. This observation has been used as a compelling argument for the use of analgesics preoperatively or intraoperatively as a preemptive strike against the development of windup during surgeries likely to activate C fibers.
Suprasegmental modulation
Activity in spinal nociceptive pathways is also strongly influenced by antinociceptive systems that originate in the brain stem. The midbrain (mesencephalon) and medulla both possess a series of midline nuclei that modulate the transmission of nociception. Input from higher cerebral centers and collaterals from ascending nociceptive pathways, particularly those conveying deep pain (spinoreticular tract), activate these nuclei. Among the nuclei that give rise to descending pain modulatory pathways, of particular note are the mesencephalic PAG and the nucleus raphe magnus of the rostroventral medulla.
The PAG receives input from ascending nociceptive tracts and higher centers (including limbic structures and cerebral cortex) and sends axons to the nucleus raphe magnus, to other medullary reticular nuclei, and, to a much lesser extent, to the dorsal horn of the spinal cord. These axons release multiple neurotransmitters, most notably endorphins, which are transmitters with powerful antinociceptive properties. The PAG input to the nucleus raphe magnus activates (through disinhibition) the monoaminergic pathways that arise here and descend the cord to modulate nociception at the level of the dorsal horn. The primary neurotransmitters of the nucleus raphe magnus and other medullary nuclei are serotonin and norepinephrine. Activity in these systems will recruit a pool of interneurons whose neurotransmitters (endorphin, enkephalin, and dynorphin) inhibit transmission in spinal cord pain pathways at the level of the dorsal horn.
This is pain that is caused by injury to the nervous system. Damage leading to neuropathic pain can result from a variety of insults, including trauma (e.g., amputation and crushing injury), vascular injury (e.g., thromboembolic disease), endocrinopathy (e.g., diabetes mellitus), or infection (e.g., postherpetic neuralgia). Neuropathic pain resulting from these many different causes is probably not a single entity. Several mechanisms are thought likely to contribute to neuropathic pain; not all of these necessarily underlie any given case of neuropathic pain, although they are not mutually exclusive.
Hyperalgesia and allodynia are both commonly associated with neuropathic pain. Dysesthesias, which are unpleasant, abnormal sensations often characterized as tingling or “electric,“ are sometimes described by affected people, although neuropathic pain is most usually described as having a burning, lancinating quality. In the peripheral nervous system, injury to primary afferents (up to and including the dorsal root ganglia) can cause neuropathic pain. The mechanisms producing this pain are not clearly understood and, like all facets of neuropathic pain, are likely to be multiple. In at least some cases, the damaged primary afferent produces an increased frequency of spontaneous APs (most neurons do this to some extent, but normal nociceptive afferents typically do so at a very low rate), a phenomenon called ectopic discharge. Damaged primary afferents are also apt to develop collateral sprouting, perhaps in response to neurotrophic factors released by damaged tissues. Aberrant collaterals of nociceptive neurons may spread into adjacent skin or other tissues, where their activation can produce an abnormal perception of pain. One line of inquiry has revealed a phenomenon of electrical coupling between somatosensory and sympathetic fibers in the periphery (including the dorsal root ganglia). This coupling, called sympathetically maintained pain, activates nociceptive pathways (and pain perception) with activity in sympathetic neurons.
Centrally, the alterations described in the discussion of windup (spinal facilitation of pain) are likely to play a role in the development of sustained, neuropathic pain, inasmuch as the upregulation of receptors on postsynaptic membranes can persist for a prolonged time. Since activation of NMDA glutamate receptors is a key part of spinal facilitation of pain, use of NMDA antagonists shows potential as a therapy for neuropathic pain. Additionally, there is evidence that tactile (i.e., nonnociceptive) A-β afferent fibers sprout collateral connections in the dorsal horn (again, probably in response to neurotrophic factors released by injured nervous tissue), making aberrant connections with projection neurons that are normally associated with nociception. Activity in these fibers will therefore produce activity in nociceptive pathways, leading ultimately to the perception of increased pain.
There is also ample evidence that the repertoire of neurotransmitters and/or receptors within the dorsal horn undergoes changes in response to injury. Some researchers have documented a decrease in γ-aminobutyric acid (GABA, an inhibitory neurotransmitter) in animal models of neuropathic pain. Experimental techniques that increase levels of GABA in the spinal cord are associated with an attenuation of allodynia, which is characteristic of neuropathic pain.
A particularly frustrating aspect of neuropathic pain is the extent to which it is refractory to opioids. This is likely because of a loss of opioid receptors, which is a phenomenon that has been reported in the dorsal root ganglion and the dorsal horn. Simultaneously, cholecystokinin and its receptors appear to be upregulated by nervous tissue injury; cholecystokinin has documented opioid antagonist activity.
Measuring pain in veterinary patients
As previously mentioned, pain is an extremely complex multidimensional experience with both sensory and affective elements. Obviously, there are distinct populations, including human neonates, nonverbal adults, and animals, that cannot express their pain overtly. However, all mammals possess the neuroanatomical and neuropharmacological components necessary for transduction, transmission, and perception of noxious stimuli; therefore, it is commonly assumed that animals experience pain even if they cannot exactly perceive or communicate it in the same way people do.
There is presently no gold standard for assessing pain in animals. Many different scoring methods that include physiological variables (in an attempt to identify objective measures) and behavioral variables have been published, but few have been rigorously validated. The issue of pain assessment in animals is especially complex because consideration must include differences in gender, age, species, breed, strain, and environment. Assessment systems must also take into account the different types and sources of pain, such as acute versus chronic or neuropathic pain and visceral compared with somatic pain. For example, if a pain scale were developed to evaluate acute postoperative pain in dogs following routine abdominal surgery, such as ovariohysterectomy, then the scale might be inappropriate for assessing pain after orthopedic surgery or pain associated with chronic osteoarthritis in that species. There is no question that as more studies focus on species-specific pain behaviors and the different types of pain, the ability of the veterinary community to recognize and treat pain in animals will improve. Nevertheless, the assessment of pain in animals will remain a subjective and inaccurate undertaking for the foreseeable future.
Behavioral responses to pain vary greatly between species, and these differences may be linked to an animal’s innate behaviors. For example, because rats and mice are prey animals, overt signs of pain or injury draw the attention of predators, so the rodents have evolved to where they instinctively disguise their pain. The subtle signs of pain exhibited by these species, such as abdominal pressing and back arching, can be easily missed by an inexperienced observer. Because of behavioral distinctions, pain assessment tools must be species specific.
Acute pain
Most studies in dogs and cats have focused on assessing acute postoperative pain. Not all of the systems used have been validated or rigorously tested, however; and the key question for a busy practitioner is “How well do these scoring systems perform in clinical practice?”
Objective measures
In both cats and dogs, the correlation between easily measured physiological variables (heart rate, respiratory rate, blood pressure, and pupil diameter) and pain scores have been evaluated. No study found a consistently reliable objective measure, which is not surprising as these parameters can be affected by many factors other than pain. For example, an opioid alone causes mydriasis in cats but miosis in dogs. Pupil size is also affected by fear and ambient light. In a tightly controlled research setting, blood pressure looked promising as an indirect indicator of pain in cats, but, in a clinical environment, this variable was an unreliable indicator of pain.
Changes in plasma cortisol and β-endorphins are components of the “stress response” to anesthesia and surgery, and much effort has been expended trying to correlate these hormones with pain in laboratory and clinical analgesia trials. Plasma cortisol was not a useful pain marker in dogs and is extremely unreliable in cats. Mechanical nociceptive threshold testing with various devices (palpometers and algometers) has proved to be useful for evaluating both primary (wound) and secondary (remote area) hyperalgesia in cats and dogs. Changes in wound sensitivity have correlated with visual analog scoring in cats, suggesting that assessing wound tenderness is a valuable tool and should be incorporated into an overall assessment protocol. Force plate gait analyses have been widely used to assess lameness in dogs objectively. This technique has also been used to evaluate response to different surgical procedures and to assess the efficacy of a variety of analgesics.
Subjective scoring systems
Because animals cannot self-report, all scoring systems that depend on a human observer must, by definition, be subjective to some degree and leave room for error, which could be either underassessment or overassessment of the animal’s pain. Any system used should be valid, reliable, and sensitive. Without strictly defined criteria and the use of well-trained and experienced observers, many scoring systems are too variable, which is one of the main criticisms of multicenter clinical trials. One scoring system may show an analgesic agent to be effective, and another shows that same analgesic to be ineffective. If a system is insensitive, then these differences are inevitable and result in large interobserver variability.
Simple descriptive scales These are the most basic pain scales. These usually have four or five descriptors from which observers choose, such as no pain, mild pain, moderate pain, severe pain, or very severe pain. Although simple to use, these scales are extremely subjective and do not detect small changes in pain behavior.
Numerical rating scales These are essentially the same as simple descriptive scales, but assign numbers for ease of tabulation and analyses; for example, absence of pain is assigned the number 0 and very severe pain the number 5. This system implies equal difference or weighting between each category, which is not the case. These are discontinuous scales; therefore, a dog experiencing pain that is “just in“ category 2 is in a quite different condition from a dog that is also in category 2 but almost in category 3. A further development of the simple descriptive and numerical rating systems is a categorized numerical rating system where certain behaviors are chosen and assigned a value. For example, vocalization can be divided into none (score = 0), crying but responsive (score = 1), and crying but nonresponsive (score = 2); other categories may include movement, agitation, and posture.
Visual analog scale In an attempt to improve on discontinuous scales, the visual analog scale (VAS) has been widely used in veterinary medicine (Figure 3.2). This tool consists of a continuous line (usually 100 mm long) anchored at either end with a description of the limits of the scale, for example no pain or no sedation at one end and severe pain or asleep at the other end. An observer places a mark on the line at the point that he/she thinks correlates with the degree of pain in the animal under observation, and this point is later translated into a number by measuring the distance to the mark from zero. Without training and experience, the VAS results in wide interobserver variation.
Dynamic and interactive visual analog scale (DIVAS) are an extension of the classic VAS system in dogs. With the DIVAS system, animals are first observed from a distance undisturbed and then approached, handled, and encouraged to walk. Finally, the surgical incision and surrounding area are palpated, and a final overall assessment of sedation and pain is made. This approach overcomes some of the deficiencies of purely observational systems; for example, a dog may lie very still and quiet because a wound is painful, and this would go undetected unless the observer interacted with the animal. The DIVAS system has also been used to assess postoperative pain in cats and, when performed by one individual unaware of treatments, it detected differences between analgesics and between treated and untreated cats. So far, the scoring systems discussed in this section are regarded as one-dimensional in that they assess only intensity of pain.
Figure 3.2. The VAS used to assess pain in animals.
Source: Hellyer P.W., Robertson S.A., Fails A.D. 2007. Pain and its management. In: Lumb and Jones’ Veterinary Anesthesia and Analgesia, 4th ed. W.J. Tranquilli, J.C. Thurmon, and K.A. Grimm, eds. Ames, IA: Blackwell Publishing, p. 43.

Considering the complexity of pain, it is not surprising that simple, subjective, onedimensional systems have not proven ideal. In humans, multidimensional systems—such as the McGill Pain Questionnaire—that account for not only intensity but also sensory and affective (emotional) qualities of pain have provided a more comprehensive assessment of a patient’s pain. Multidimensional systems are particularly important when self-reporting is not possible, but reports must incorporate components that are proven to be sensitive and specific to pain (e.g., facial expressions in infants) in the species being studied.
The University of Melbourne Pain Scale (UMPS) has been developed to incorporate objective physiological data (heart rate, respiratory rate, pupil size, and rectal temperature) and behavioral responses (activity, response to palpation, posture, mental status, and vocalization). By assigning numbers to each factor, a score between 0 and 27 is derived. This scale has been tested on dogs following ovariohysterectomy and demonstrated good agreement between different assessors. It could differentiate between dogs that were anesthetized but not subjected to surgery and those undergoing surgery. With some refinement to detect smaller differences, the system shows promise for clinical use.
To date, the most vigorously validated scale for assessing acute postoperative pain in dogs is the Glasgow Composite Measures Pain Scale. The original 279 words or expressions that could describe pain in dogs have been reduced to 47 well-defined words placed in one physiological category and seven behavioral categories. The behavioral categories comprise evaluations of: posture, comfort, vocalization, attention to the wound, demeanor and response to humans, mobility, and response to touch. Each descriptor is well defined to avoid misinterpretation. Assessment involves both observation from a distance and interaction with the patient (e.g., palpation of the wound). Frequent assessments are necessary because pain is not a static process, and the benefits of intervention with analgesics must be evaluated. In a busy practice, time-consuming assessments are the biggest drawbacks to effective pain management. For this reason, a short form of the Glasgow composite pain scale, which takes only a few minutes to perform, has been developed (Figure 3.3).
How often should animals be assessed
The health status of the animal, extent of surgery/injuries, and anticipated duration of analgesic drugs determine the frequency and interval of evaluations. In general, evaluations should be made at least hourly for the first 4–6 hours after surgery, provided the animal has recovered from anesthesia, has stable vital signs, and is resting comfortably. Animals not recovering as anticipated from anesthesia/surgery and critically ill animals require much more frequent evaluations until they are stabilized. Patient response to analgesic therapy and expected duration of analgesic drug(s) administered help to determine frequency of evaluations. For example, if a dog is resting comfortably following the postoperative administration of morphine, it may not need to be reassessed for 2–4 hours. Animals should be allowed to sleep following analgesic therapy. Vital signs can often be checked without unduly disturbing a sleeping animal. In general, animals are not awakened to check their pain status; however, that does not mean they should not receive their scheduled analgesics.
Continuous, undisturbed observations, coupled with periodic interactive observations (open the cage, palpate the wound, etc.) are likely to provide more information than occasionally observing the animal through the cage door. It is regrettable that continuous observations are not practical for most clinical situations. In general, the more frequent the observations, the more likely that subtle signs of pain will be detected.
Chronic pain
Chronic pain can affect an animal‘s quality of life. Because of the nature of chronic pain, such as that associated with osteoarthritis in dogs and cats, the accompanying behavioral changes can be insidious and easily missed. Indeed, many owners assume these changes are inevitable with advancing age. Preliminary data based on owner interviews revealed changes in 32 types of behavior in dogs with chronic pain. This study also indicated that the owners are the best evaluators of their pet’s pain. The Glasgow University HealthRelated Dog Behavior Questionnaire has identified some key indicators of chronic pain, including, but not limited to, decreases in mobility, activity, sociability, and curiosity, and increases in aggression, anxiety, daytime sleeping, and vocalizing.
Figure 3.3. The short-form composite measure pain score (CMPS-SF) can be applied quickly and reliably in a clinical setting and has been designed as a clinical decision-making tool that was developed for dogs in acute pain. It includes 30 descriptor options within six behavioral categories, including mobility. Within each category, the descriptors are ranked numerically according to their associated pain severity and the person carrying out the assessment chooses the descriptor within each category that best fits the dog’s behavior/condition. It is important to carry out the assessment procedure as described on the questionnaire, following the protocol closely. The pain score is the sum of the rank scores. The maximum score for the six categories is 24, or 20 if mobility is impossible to assess. The total CMPS-SF score has been shown to be a useful indicator of analgesic requirement and the recommended analgesic intervention level is 6/24 or 5/20.
Source: Reproduced with the permission of Jacky Reid, Professor of Veterinary Anaesthesia, University of Glasgow.

Chronic pain is undoubtedly a clinical problem in cats, but is not well documented. Compared with dogs, very little is known about degenerative joint disease in cats, but radiographic evidence in geriatric cats suggests the incidence may be as high as 90%. Because of a pet cat‘s lifestyle, lameness is not a common owner complaint; but changes in behavior, including decreased grooming, reluctance to jump up to favorite places, and soiling outside the litter box, should prompt veterinarians to look for sources of chronic pain. It is common for owners not to realize how debilitated their pet is until they see dramatic improvements following treatment.
Analgesia in the strictest sense is an absence of pain but clinically is the reduction in the intensity of pain perceived (hypoalgesia). The goal should not be to eliminate pain completely, but to make the pain as tolerable as possible without undue depression of patients. Analgesia in the clinical setting may be induced by obtunding or interrupting the nociceptive process at one or more points between the peripheral nociceptor and the cerebral cortex.
Nociception involves four physiological processes that are subject to pharmacological modulation. Transduction is the translation of physical energy (noxious stimuli) into electric activity at the peripheral nociceptor. Transmission is the propagation of nerve impulses through the nervous system. Modulation occurs through the endogenous descending analgesic systems, which modify nociceptive transmission. These endogenous systems (opioid, serotonergic, and noradrenergic) modulate nociception through inhibition of the spinal dorsal horn cells. Perception is the final process resulting from successful transduction, transmission and modulation, and integration of thalamocortical, reticular, and limbic function to produce the final conscious subjective and emotional experience of pain.
Transduction can be largely abolished by use of local anesthetics infiltrated at the site of injury or incision, or by intravenous, postthoracotomy intrapleural, or postlaparotomy intraperitoneal injection.
Nonsteroidal anti-inflammatory drugs (NSAIDs) will obtund transduction by decreasing production of endogenous algogenic substances such as PGs at the site of injury. Transmission can be abolished by local anesthetic blockade of peripheral nerves or nerve plexuses or by epidural or subarachnoid injection. Modulation can be augmented by subarachnoid or epidural injection of opioids, and/or alpha2 adrenergic agonists. Perception can be obtunded with general anesthetics or by systemic administration of opioids and alpha2 agonists, either alone or in combination with tranquilizer-sedatives.
Balanced or multimodal analgesia results from the administration of analgesic drugs in combination and at multiple sites to induce analgesia by altering more than one part of the nociceptive process. Multimodal analgesia relies on the additive or synergistic effects of two or more analgesic drugs working through different mechanisms of action. When multimodal analgesia is used, doses of individual drugs can usually be reduced, thereby theoretically decreasing the potential for any one drug to induce adverse side effects.
Preemptive analgesia refers to the application of balanced analgesic techniques prior to exposing patients to noxious stimuli (surgical trespass). By so doing, the spinal cord is not exposed to the barrage of afferent nociceptive impulses that induce the neuroplastic changes leading to central hypersensitivity. By consensus, this concept has gained acceptance as the most effective means of controlling postoperative pain.
There are three major classes of analgesic agents employed in veterinary medicine for the management of pain: opioids, NSAIDs, and local anesthetics. In addition to these three traditional drug classes, another diverse group of agents used to manage pain is known collectively as analgesic adjuvants.
Opioids
All opioid analgesics are chemically related to a group of compounds that have been purified from the juice of a particular species of poppy: Papaverum somniferum. The unrefined extract from the poppy is called opium and contains approximately 20 naturally occurring pharmacologically active compounds, including familiar ones like morphine and codeine. This group of purified natural agents is specifically referred to as opiates. In addition, numerous semisynthetic and synthetic analogs of the opiates have been developed for clinical use. The word opioid is used broadly to cover all drugs that are chemical derivatives of the compounds purified from opium and is the term that is used throughout this chapter.
The opioids continue to be the cornerstone of effective pain treatment in veterinary medicine. They are a versatile group of drugs with extensive applications in the management of pain in patients with acute trauma, in patients undergoing surgical procedures, in patients with painful medical conditions or disease processes, and in patients suffering from chronic pain that require long-term therapy.
It is well known that exogenously administered opioids such as morphine exert their effects by interacting with specific opioid receptors and mimicking naturally occurring molecules known as endogenous opioid peptides. There are three well-defined types of opioid receptors, most commonly known by their Greek letter designations as μ (mu), δ (delta), and κ (kappa). This classic system of nomenclature has been under reconsideration for a number of years and, during this time, several alternative naming systems have been proposed, leading to considerable confusion. In addition, a fourth type of opioid receptor, the nociceptin receptor (also known as the orphanin FQ receptor), has been characterized. According to the most recent recommendations of the International Union of Pharmacology Subcommittee on Nomenclature, variations based on the Greek letters remain acceptable. Thus, mu, μ, or MOP (for mu opioid peptide); delta, δ, or DOP (for delta opioid peptide); kappa, κ, or KOP (for kappa opioid peptide); and NOP (for nociceptin opioid peptide) are considered interchangeable abbreviations. Distinct complementary DNA (cDNA) sequences have been cloned for all four opioid receptor types, and each type appears to have a unique distribution in the brain, spinal cord, and periphery.
The diversity of opioid receptors is further extended by the existence of several subtypes of μ, δ, and κ receptors. Based on pharmacological studies, there are thought to be at least three μ-receptor subtypes, μ1, μ2, and μ3; two δ-receptor subtypes, δ1 and δ2; and perhaps as many as four κ-receptor subtypes, κ1a, κ1b, κ2, and κ3. The discovery of opioid receptor subtypes generated great enthusiasm among researchers and introduced the possibility of developing subtype-specific therapeutic agents with favorable sideeffect profiles. At this point, however, the functional significance of these receptor subtypes remains unclear, and distinct cDNA sequences corresponding to these subtypes have not yet been identified.
In general, it appears that the μ receptor mediates most of the clinically relevant analgesic effects, as well as most of the adverse effects associated with opioid administration. Drugs acting at the δ receptor tend to be poor analgesics, but may modify μ receptormediated antinociception under certain circumstances and mediate opioid receptor “cross-talk.” The κ receptor mediates analgesia in several specific locations in the CNS and the periphery, but distinguishing μ-and κ-mediated analgesic effects has proven to be difficult.
In contrast to the classic opioid receptors, the nociceptin receptor does not mediate typical opioid analgesia, but instead produces antiopioid (pronociceptive) effects. Because of the considerable structural homology among the three classically described opioid receptors, it is likely that there are significant interactions among these receptors in different tissues, and the loosely defined physiological roles ascribed to each receptor type still require further clarification.
Endogenous receptor ligands
The aforementioned opioid receptors discussed are part of an extensive opioid system that includes a large number of endogenous opioid peptide ligands. Endogenous opioid peptides are small molecules that are naturally produced in the CNS and in various glands throughout the body, such as the pituitary and the adrenal. Three distinct families of endogenous opioid peptides have been identified: the enkephalins, the dynorphins, and β endorphin. Each of these is derived from a distinct precursor polypeptide: proenkephalin, prodynorphin, and proopiomelanocortin, respectively. These endogenous opioid peptides are expressed throughout the CNS, and their presence has been confirmed in peripheral tissues, as well. There are considerable structural similarities among these three groups of peptides, and each family demonstrates variable affinities for μ, δ, and κ receptors. None of them bind exclusively to a single opioid receptor, and none of them have any significant affinity for the nociceptin receptor. The physiological roles of these peptides are not completely understood at this time. They appear to function as neurotransmitters, neuromodulators, and, in some cases, as neurohormones. They mediate some forms of stress-induced analgesia and also play a role in analgesia induced by electrical stimulation of discrete regions in the brain, such as the periaqueductal gray area of the mesencephalon.
Nociceptin (also known as orphanin FQ) is the endogenous ligand for the more recently discovered nociceptin receptor. Nociceptin is derived from pronociceptin, and its amino acid sequence is closely related to that of the aforementioned endogenous opioid peptides. Despite this homology, nociceptin binding is specific for the nociceptin receptor, and the peptide does not appear to interact with μ, δ, or κ receptors. Furthermore, the physiological effects of nociceptin are in direct contrast to the actions of the classical endogenous opioid peptides, with nociceptin producing a distinctly pronociceptive effect. The functional significance of nociceptin and its receptor remains to be elucidated, but additional insight into this novel opioid peptide may have substantial implications in future therapeutic drug development.
In addition to the enkephalins, dynorphins, β-endorphin, and nociceptin, there are now two other recently discovered endogenous opioid peptides called endomorphin 1 and endomorphin 2. These peptides are putative products of an as yet unidentified precursor and have been proposed to be the highly selective endogenous ligands for the μ receptor. The endomorphins are small tetrapeptides that are structurally unrelated to the endogenous opioid peptides. Their identification has heralded a new era in research of the μ opioid system, which may contribute to our understanding of the neurobiology of opioids and provide new avenues for therapeutic interventions.
Signaling and mechanisms of analgesia
Binding of an opioid agonist to a neuronal opioid receptor, regardless of whether the agonist is endogenous or exogenous, typically leads to several events that serve to inhibit the activation of the neuron. Opioid receptors are part of a large superfamily of membrane bound receptors that are coupled to G proteins. As such, they are structurally and functionally related to receptors for many other neurotransmitters and neuropeptides that act to modulate the activity of nerve cells. Opioid receptor binding, via activation of various types of G proteins, may inhibit adenylyl cyclase (cyclic adenosine monophosphate) activity, activate receptor-operated potassium ion (K+) currents, and suppress voltagegated calcium ion (Ca2+) currents.
At the presynaptic level, decreased Ca2+ influx will reduce release of transmitter substances, such as substance P, from primary afferent fibers in the spinal cord dorsal horn, thereby inhibiting synaptic transmission of nociceptive input. Postsynaptically, enhanced K+ efflux causes neuronal hyperpolarization of spinal cord projection neurons and inhibits ascending nociceptive pathways. A third potential mode of opioid action involves upregulation of supraspinal descending antinociceptive pathways in the PAG. It is now known that this system is subject to tonic inhibition mediated by GABAergic neurons, and opioid receptor activation has been shown to suppress this inhibitory influence and augment descending antinociceptive transmission. The proposed cellular basis for this involves μ receptors that activate voltage-dependent K+ ions present on presynaptic GABAergic nerve terminals that inhibit GABA release into the synaptic cleft. It is important to note that although our collective understanding of opioid receptor-mediated signaling has increased dramatically in recent years, the relationship of such subcellular events to clinical analgesia at the level of the organism continues to require further clarification.
Distribution and therapeutic implications
Although cellular and molecular studies of opioid receptors and ligands are invaluable in understanding their function, it is critical to place opioid receptors in their anatomical and physiological context to fully appreciate the opioid system and its relevance to pain management. It has long been a principle tenet of opioid analgesia that these agents are centrally acting, and this understanding has shaped the way we use opioid analgesics clinically. It has been well established that the analgesic effects of opioids arise from their ability to directly inhibit the ascending transmission of nociceptive information from the spinal cord dorsal horn, and to activate inhibitory pathways that descend from the midbrain via the rostral ventromedial medulla to the spinal cord. Within the CNS, evidence of μ, δ, and κ opioid receptor messenger RNA and/or opioid peptide binding has been demonstrated in supraspinal sites, including the mesencephalic PAG, the mesencephalic RF, various nuclei of the rostral ventromedial medulla, and forebrain regions including the nucleus accumbens, as well as spinally within the dorsal horn. The interactions between groups of opioid receptors at various spinal and supraspinal locations, as well as interactions among different receptor types within a given location are complex and incompletely understood at this time.
Systemic administration of opioid analgesics via intravenous, intramuscular, or subcutaneous injection will induce a relatively rapid onset of action via interaction with these CNS receptors. Oral, transdermal, rectal, or buccal mucosal administration of opioids will result in variable systemic absorption, depending on the characteristics of the particular agent, with analgesic effects being mediated largely by the same receptors within the CNS. In addition, neuraxial administration, either into the subarachnoid or epidural space, is an efficacious route of administration. Small doses of opioids introduced via these routes readily penetrate the spinal cord and interact with spinal and/or supraspinal opioid receptors to produce profound and potentially long-lasting analgesia, the characteristics of which will depend on the particular drug used.
Even though opioids have long been considered the prototype of centrally acting analgesics, a body of evidence has emerged that clearly indicates that opioids can produce potent and clinically measurable analgesia by activation of opioid receptors in the peripheral nervous system. Opioid receptors of all three major types have been identified on the processes of sensory neurons, and these receptors respond to peripherally applied opioids and locally released endogenous opioid peptides when upregulated during inflammatory pain states. Furthermore, although sympathetic neurons and immune cells have also been shown to express opioid receptors, their functional role remains unclear. Although the binding characteristics of peripheral and central opioid receptors are similar, the molecular mass of peripheral and central μ opioid receptors appears to be different, suggesting that selective ligands for these peripheral receptors could be developed that would produce opioid analgesia without the potential to induce centrally mediated adverse side effects.
Adverse effects
Although opioids are used clinically primarily for their pain-relieving properties, they also produce a host of other effects on a variety of body systems. This is not surprising in light of the wide distribution of endogenous opioid peptides and their receptors in supraspinal, spinal, and peripheral locations. Some of these adverse effects, such as sedation, may be classified as either desirable or undesirable depending on the clinical circumstances. The following is a brief summary of these major side effects as they relate to opioids as a class of drugs.
CNS
There are considerable species differences in the CNS response to opioid analgesics that cannot be attributed to pharmacokinetic variations alone. CNS depression (i.e., sedation) is typically seen in dogs, monkeys, and people, whereas CNS stimulation (i.e., excitement and/or spontaneous locomotor activity) may be elicited in cats, horses, goats, sheep, pigs, and cows after systemic administration of various opioids, most notably morphine. Reasons for these different responses are not entirely clear at this time, but are presumably related to differing concentrations and distributions of μ, δ, and κ receptors in various regions of the brain in these species. Despite these fundamental differences, it must be remembered that there are numerous factors that may affect the CNS response to opioids within a given species, including the temperament or condition of the patient; the presence or absence of pain; the dose, route, and timing of drug administration; and the specific opioid administered.
The hypothalamic thermoregulatory system is also affected by opioid administration. Hypothermia tends to be the most common response, particularly when opioids are used during the perioperative period in the presence of other CNS-depressant drugs. Under some clinical circumstances, however, opioid administration causes hyperthermia in cats. Part of this increase in body temperature may be attributed to an increase in muscle activity associated with CNS excitation in this species; however, a specific central hypothalamic mechanism has also been implicated, but remains poorly understood. Panting is seen commonly after opioid administration, most often in dogs, but this effect tends to decrease with the onset of hypothermia.
Nausea and vomiting associated with opioid administration are caused by direct stimulation of the chemoreceptor trigger zone for emesis located in the area postrema of the medulla. As with the other centrally mediated side effects, species plays a role in determining an individual‘s tendency to vomit after an opioid is administered. Cats may vomit, but usually at doses that are greater than those which stimulate vomiting in dogs. Dogs will commonly vomit after opioid administration, especially with morphine. Emesis is rarely seen when opioids are administered in the immediate postoperative period or in any patient that may be experiencing some degree of pain.
Opioids have variable efficacy in depressing the cough reflex, at least in part by a direct effect on a cough center located in the medulla. Certain opioids are more effective antitussives than others, and drugs like codeine, hydrocodone, and butorphanol are occasionally prescribed specifically for this indication.
As a general rule, opioids tend to produce mydriasis in those species that exhibit CNS excitation, and miosis in those that become sedated after opioid administration. Miosis is produced by an excitatory action of opioids on neuronal firing in the oculomotor nucleus. In cats, and presumably in other species that exhibit mydriasis, this increase in activity in the oculomotor nuclear complex still occurs, but the miotic effect is masked by increased release of catecholamines, which produces mydriasis.
Respiratory system
Opioids produce dose-dependent depression of ventilation, primarily mediated by μ2 receptors, leading to a direct depressant effect on brain stem respiratory centers. This effect is characterized by decreased responsiveness of these centers to carbon dioxide and is reflected in an increased resting arterial carbon dioxide partial pressure and displacement of the carbon dioxide response curve to the right. This effect is compounded by the coadministration of sedative and/or anesthetic agents, meaning that significant respiratory depression and hypercapnia are much more likely to occur in anesthetized patients that receive opioids compared with those that are conscious. It should be noted that, in general, humans tend to be more sensitive to the respiratory depressant effects of opioids when compared with most veterinary species, and the risk of hypoventilation would rarely constitute a legitimate reason for withholding opioid treatment in clinical practice; however, careful patient monitoring is prudent.
Cardiovascular system
Most opioids have minimal effects on cardiac output, cardiac rhythm, and arterial blood pressure when clinically relevant analgesic doses are administered. Bradycardia may be caused by opioid-induced medullary vagal stimulation and will respond readily to anticholinergic treatment. Particular opioids (morphine and meperidine) can cause histamine release, especially after rapid intravenous administration, which may lead to vasodilation and hypotension. Because of their relatively benign effects on cardiovascular function, opioids commonly form the basis of anesthetic protocols for patients with preexisting cardiovascular disease.
Gastrointestinal system
The gastrointestinal effects of the opioids are mediated by μ and δ receptors located in the myenteric plexus of the gastrointestinal tract. Opioid administration will often stimulate dogs and, less frequently, cats to defecate. After this initial response, spasm of gastrointestinal smooth muscle predisposes patients to ileus and constipation. These side effects tend to be most significant with prolonged administration of opioids in dogs and cats experiencing chronic pain, and such patients may require dietary modifications and stool-softening medications to manage these adverse effects.
In human patients, opioids (most notably fentanyl and morphine) have been shown to increase bile duct pressure through constriction of the sphincter of Oddi. The incidence of this side effect in people is, however, quite low. Despite anatomical differences, this observation has led to concerns about opioid administration to dogs and cats with pancreatitis and/or cholangitis. A study reviewing the body of human literature found that, despite widespread clinical practice, there was no evidence to indicate that morphine is contraindicated for use in acute pancreatitis. As there are no studies that specifically evaluate the effects of opioids in dogs and cats with pancreatitis, it does not at this time seem appropriate to withhold this class of drugs from this subset of severely painful patients.
Genitourinary system
Opioids, particularly when administered neuraxially, may cause urinary retention through dose-dependent suppression of detrusor contractility and decreased sensation of urge. Manual expression of the urinary bladder or catheterization may be required in certain individuals until urodynamic function returns to normal. Urine volume may also be affected by opioids, and the mechanism of this effect appears to be multifactorial. μ-Agonists tend to produce oliguria in the clinical setting, and this is in part due to increased antidiuretic hormone release leading to altered renal tubular function. Elevations in circulating plasma atrial natriuretic peptide may also play a role in morphine-induced antidiuresis. Conversely, κ-agonists tend to produce a diuretic effect, possibly through inhibition of antidiuretic hormone secretion. Other peripheral mechanisms involving stimulation of renal alpha2 adrenergic receptors may also contribute to this κ-agonist effect.
Agonists
Almost all clinically useful opioids exert their analgesic effects by acting as agonists at μ receptors. Although a few opioids act as κ-agonists, these drugs also tend to have antagonist or partial agonist effects at μ and/or δ receptors and are thus not classified as pure agonists. Pure or full opioid agonists can elicit maximal activation of the receptor when they bind it, and the subsequent downstream processes produce a maximal analgesic effect (Figure 3.4). Clinically, the full μ-agonists are superior analgesics and are the drugs of choice for pain of moderate to severe intensity in many veterinary species (see Table 3.1 for recommended dosages).
Figure 3.4. A lock-and-key analogy is used to illustrate full agonist drug interactions at opioid receptors, with a relative dose-response curve for analgesic effectiveness shown. A full opioid agonist (in this example morphine) stimulates both and K-receptor types, which produces increased analgesic effect with increased dose.
Source: Modified from Nicholson A., Christie M. 2002. Opioid analgesics. In: Small Animal Clinical Pharmacology. J. Maddison, S. Page, and D.B. Church, eds. Philadelphia, PA: WB Saunders, pp. 271–292.

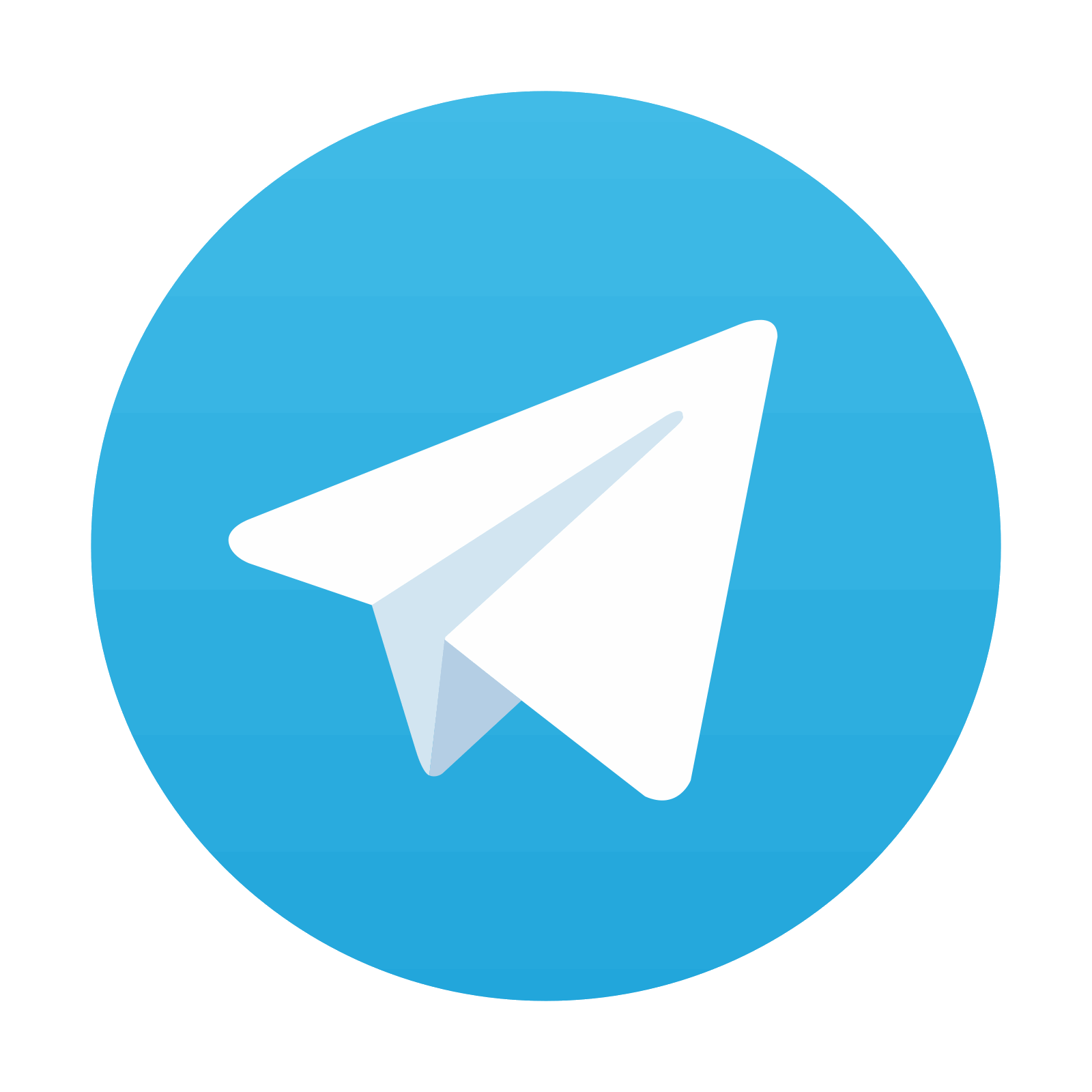
Stay updated, free articles. Join our Telegram channel
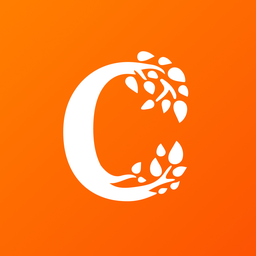
Full access? Get Clinical Tree
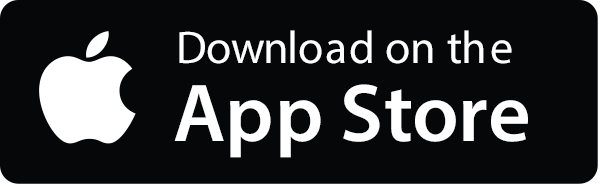
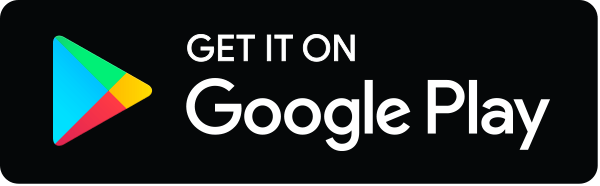