Francisco J. Teixeira‐Neto1 and Carolyn L. Kerr2 1 Faculdade de Medicina Veterinária e Zootecnia, Universidade Estadual Paulista (UNESP), Botucatu, Brazil 2 Department of Clinical Studies, Ontario Veterinary College, University of Guelph, Guelph, Ontario, Canada Sedation and general anesthesia can produce profound changes in respiratory function, with the degree of change depending on drugs employed, species involved, depth of anesthesia, surgical procedure, and the health of the individual animal. Of greatest concern is significant reduction in gas exchange causing inadequate oxygen delivery to vital tissues and/or carbon dioxide (CO2) removal from the body. The aim of oxygen supplementation/therapy is to increase the alveolar oxygen partial pressure (PAO2) to a level that achieves a target arterial hemoglobin oxygen saturation (SaO2), most commonly > 94%. This objective is achieved by increasing the inspired oxygen fraction (FIO2) in both intubated and non‐intubated patients. Oxygen supplementation may be necessary before induction of general anesthesia, during anesthesia, and into the postanesthetic period. To prevent a decrease in SaO2/hypoxemia caused by the decreased minute ventilation (V̇E) induced by anesthetic drugs, it is common practice to increase the FIO2 in spontaneously breathing patients. However, in animal species where atelectasis plays a major role in causing oxygenation impairment during anesthesia (e.g., horses), use of a high FIO2 (> 0.8) may not abolish or prevent the development of hypoxemia [1,2]. The use of mechanical ventilators to provide positive‐pressure ventilatory support has become a routine component of anesthesia management in both small and large animal referral hospitals. While mechanical ventilatory support has variable effects on PaO2 in animals with respiratory disease or in the presence of significant atelectasis, it is an effective means of preventing the increase in arterial carbon dioxide partial pressure (PaCO2) caused by anesthetic drugs. Supporting ventilation by an artificial means, in addition to counteracting the negative effects of anesthesia on gas exchange, will also decrease the work of breathing, which may be particularly beneficial in animals presenting with clinical conditions associated with fatigue of respiratory muscles [3]. Mechanical ventilation is an essential support measure for patients that are undergoing intrathoracic procedures including thoracoscopy, thoracotomy, and/or one‐lung ventilation (OLV). It is important for the clinician to understand the physiologic effects of oxygen therapy and mechanical ventilation, in addition to their indications and contraindications, to maximize their benefits and minimize any potential negative effects associated with their use. In addition to standard perianesthetic patient preparation, use of balanced anesthetic techniques, monitoring, and supportive care, specific considerations for managing patients with underlying respiratory disease that require anesthesia for a variety of procedures are discussed below. Inspired oxygen supplementation is routinely performed in both the perianesthetic period and intensive care setting. Overall, oxygen therapy aims to prevent hypoxemia by maintaining arterial hemoglobin oxygen saturation above 94%. Hypoxemia refers to an abnormally low PaO2 or SaO2 compromising the oxygen content in arterial blood (CaO2), which may lead to an inadequate oxygen supply to tissues. Animals may be considered hypoxemic when PaO2 levels are < 80 mmHg and SaO2 is < 95% [4]. Oxygen supplementation to maintain hemoglobin saturation above 90% (or PaO2 > 60 mmHg) may be acceptable (assuming normal hemoglobin levels) as the CaO2 will be close to values observed when hemoglobin is fully saturated. Depending on the species and health of the individual animal, oxygen supplementation may be required prior to, during, or following anesthesia to prevent hypoxemia. Supplementation of inspired gases with oxygen, either alone or with ventilatory support, results in an increase in PAO2, as described by the alveolar gas equation (Chapter 37, Fig. 37.2, and Table 37.5). The impact of altering FIO2 on PAO2 with varying levels of alveolar ventilation is highlighted in Fig. 38.1. If alveolar ventilation is decreased by 50% of its normal resting value, PAO2 in an individual breathing ambient air at sea level is predicted to decrease from values close to 100 mmHg to < 50 mmHg (effect related to CO2 build‐up within the alveoli). However, if FIO2 is increased from 0.21 (ambient air) to values between 0.3 and 0.4 (or more), alveolar oxygen will be maintained above 100 mmHg despite severe hypoventilation. When matching of alveolar ventilation and perfusion (V̇A/Q̇) within the lung is close to ideal, minimal V̇A/Q̇ mismatch (lung areas with low V̇A/Q̇ ratio) or intrapulmonary shunt (V̇A/Q̇ ratio = 0) are present, and the alveolar–capillary membrane is normal, an increase in PAO2 induced by enriching the inspired oxygen concentration will significantly raise PaO2 as illustrated in Fig. 38.2. Although increasing FIO2 and PAO2 can be an effective means of preventing hypoxemia caused by hypoventilation, V̇A/Q̇ mismatch, and diffusion impairment (thickening of the alveolar–capillary barrier), its effects on hypoxemia caused by intrapulmonary shunt are dependent on the percentage of mixed‐venous blood that bypasses ventilated lung areas, specifically, the shunt fraction (Q̇s/Q̇T). As there is a progressive increase in Q̇s/Q̇T, the gradient between alveolar and arterial oxygen partial pressures (P(A‐a)O2) increases to the point where high percentages of shunted flow (≥ 30%) results in little or no improvement in PaO2 in response to inspired oxygen concentrations approaching 100% [6]. Therefore, whenever progressive increases in FIO2 fail to improve arterial oxygenation, a major increase in shunt flow should be suspected and the possible causes investigated and treated. Anesthetized healthy dogs and cats typically have relatively low levels of venous admixture (< 10%) originating from lung areas with a low V̇A/Q̇ ratio and from intrapulmonary shunting (V̇A/Q̇ = 0), therefore, oxygen supplementation results in PaO2 values approaching theoretical PAO2 levels. Studies evaluating the respiratory effects of inhalant or injectable anesthetics in spontaneously breathing dogs and cats receiving oxygen (FIO2 > 0.9) have reported PaO2 levels greater than 450 mmHg that remain constant over time periods typical of clinical anesthesia [7–13]. Although PaO2 values ≥ 450 mmHg result in 100% SaO2, these values are less than the predicted PAO2 (> 600 mmHg) probably because of normal physiologic shunt (bronchial veins and myocardial thebesian veins that bypass the lung) as well as shunted flow in some alveolar units due to atelectasis. Experimental research has shown that the use of FIO2 of 0.4 during anesthesia in small animals reduced atelectasis in comparison to an FIO2 > 0.9 [14,15]. The impact of different FIO2s on the development of atelectasis over time in healthy dogs and cats has yet to be demonstrated. Figure 38.1 Effect of varying FIO2 levels with various degrees of alveolar hypoventilation and hyperventilation on alveolar oxygen partial pressure (PAO2) at sea level (barometric pressure of 760 mmHg). Alveolar ventilation is displayed on the x‐axis with N representing normal alveolar ventilation and PAO2 is on the y‐axis (see text for explanation). Source: Modified from Nunn [5], reproduced with permission of Elsevier. Figure 38.2 An isoshunt diagram depicting the relationship between inspired oxygen concentration, PaO2, and various degrees of venous admixture or pulmonary shunt. Shunt flow is expressed as a percentage of cardiac output, ranging from 0 to 50%. The arteriovenous (a‐ Source: Redrawn from Benatar et al. [6], with permission. During procedures performed under deep sedation or with short‐duration intravenous anesthesia, a minimum FIO2 of 0.3 (which can be easily achieved by using flow‐by oxygen or by supplementing oxygen through a face mask) is recommended to minimize the risk of hypoxemia due to hypoventilation and V̇A/Q̇ mismatching. Evidence to support this recommendation includes reports of impaired oxygenation as reflected by PaO2 values below 65 mmHg in dogs sedated with an α2‐adrenergic receptor agonist, either alone or in combination with an opioid, in addition to reports of cyanosis and low PaO2 values during induction of anesthesia with injectable anesthetics when supplemental oxygen is not provided [16–19]. In some animals receiving oxygen supplementation, PaO2 may not increase as predicted based on the delivered FIO2. Small animal patients with pulmonary parenchymal disease typically have clinically significant alveolar–capillary diffusion barriers, V̇A/Q̇ mismatching, and increased shunt fraction. These patients may have lower‐than‐expected increases in PaO2 associated with oxygen therapy [20–23]. Recumbency and general anesthesia of mature large animal patients commonly produce significant V̇A/Q̇ mismatch and intrapulmonary shunting of blood due to anesthesia‐related atelectasis [1,24]. Thus, despite normal lung parenchyma prior to anesthesia, these patients have a reduced and variable response to oxygen supplementation when recumbent. Horses breathing ambient air with an intravenous anesthetic regimen commonly have PaO2 values in the range of 50–70 mmHg during recumbency [25,26]. During inhalational anesthesia with 100% oxygen as the carrier gas, laterally recumbent spontaneously breathing healthy horses have PaO2 values that typically range from 300 to 350 mmHg; horses in dorsal recumbency often have lower levels, ranging from 200 to 300 mmHg [2]. Unlike the dog and cat, where PaO2 levels usually remain stable over time during general anesthesia with an FIO2 > 0.95, PaO2 values often decrease over time in the equine, particularly when they are positioned in dorsal recumbency [2,27–30]. While oxygen supplementation is generally administered to reduce the risk of hypoxemia, high FIO2 during general anesthesia (to either spontaneously breathing or mechanically ventilated patients) can contribute to atelectasis in numerous species, including dogs, cats, horses, and humans [14,15,31–34]. Absorption of oxygen, which is more soluble than nitrogen, into the bloodstream and out of the alveoli, is responsible for the atelectasis associated with high inspired FIO2. If the degree of atelectasis resulting from absorption is significant, it can contribute to an increase in the P(A‐a)O2 gradient. In larger species such as the horse, the contribution of absorption of alveolar gases relative to the development of atelectasis because of compression of alveoli by abdominal contents may be minor, but its significance may vary with body position and the specific inspired oxygen level [24,27,35,36]. In humans, atelectasis attributed to high FIO2 values has been associated with an increased incidence of postoperative hypoxemia and respiratory complications [37]. The presence of atelectasis has been associated with progression of lung injury in human patients and in numerous experimental animal models of lung injury receiving ventilatory support (see below). In veterinary species, the clinical significance of anesthesia‐associated atelectasis without hypoxemia on lung health in the patient with no pre‐existing pulmonary disease is yet undetermined. Irrespective of the impact on the creation or progression of lung injury, the presence of atelectasis may affect the quality of thoracic imaging and may warrant consideration when evaluating and comparing images to those obtained while the animal is exposed to a lower FIO2 or following application of specific ventilator strategies aimed at maintaining lung volume [38]. In humans, administration of 100% oxygen via face mask prior to and during the induction of general anesthesia (i.e., preoxygenation) has been a relatively standard procedure to increase the time to hemoglobin desaturation once oxygen is withdrawn, as occurs during orotracheal intubation. However, this technique is now being called into question as it has been shown to result in a greater degree of atelectasis compared with inspiring lower oxygen fractions such as 60 or 80% [33]. Although preoxygenation is not always accomplished in healthy veterinary patients, it is recommended in cases where a prolonged time from induction of anesthesia to securing an airway is anticipated, or in animals with respiratory disease. Relative to the situation in human anesthesia, when oxygen is administered via a non‐sealed face mask to veterinary species in the clinical setting, it is unlikely that FIO2 values will be greater than 0.8 unless an unusually tight‐fitting face mask is utilized [39,40]. Spontaneously breathing anesthetized patients receiving oxygen supplementation may experience higher PaCO2 levels than animals breathing either room air or an FIO2 < 0.5 [27,31,39,41]. Horses anesthetized with halothane receiving an FIO2 of 0.3 versus > 0.85 had significantly lower PaCO2 levels [27]. Similar findings have been reported in horses under injectable anesthesia [31]. Dogs that were preoxygenated prior to induction of anesthesia also had a tendency toward an increased PaCO2 compared with dogs breathing room air [39]. Maintenance of PaO2 above levels that increase peripheral chemoreceptor input into the respiratory center in the brainstem (PaO2 > 60–70 mmHg) is likely the main mechanism responsible for a greater degree of hypoventilation in animals receiving oxygen supplementation compared to animals breathing a lower FIO2 during anesthesia. In intubated patients connected to an anesthetic machine equipped with oxygen and air flowmeters, the flow rate of gases entering the breathing circuit can be adjusted to achieve a variable FIO2 (e.g., 0.4). Most veterinary anesthesia machines, however, do not permit adjustment of FIO2 because they are equipped with only an oxygen flowmeter and therefore deliver an FIO2 > 0.95 in an animal with a sealed airway (for example, with an endotracheal tube or laryngeal mask in place). In non‐intubated patients, there are numerous different strategies/techniques which are described below that are used to increase a patient’s FIO2. In conscious, sedated, or anesthetized non‐intubated patients, FIO2 can be increased above 0.3 by administering oxygen at the level of the nares, nasopharynx, or trachea. Oxygen supplementation in non‐intubated patients is commonly provided using low‐flow systems that deliver a maximum oxygen flow rate of 15 L/min. These systems include (1) flow‐by oxygen near the nostrils/mouth; (2) supply of oxygen by face mask; and (3) supply of oxygen by nasal, nasopharyngeal, or nasotracheal catheter. The efficacy of these systems in increasing FIO2 will be dependent on the amount of dilution of the inspired gases with room air. For example, in animals that present with tachypnea, the inspiratory flow may greatly exceed the flow rate provided by the external oxygen source. As a result, the FIO2 will be less than expected because ambient air will enter the lungs to a greater degree when compared to an animal that is breathing at a slower respiratory rate, a phenomenon referred to as “air entrainment” [42]. In animals receiving oxygen supplementation through a nasal catheter, dilution of inspired oxygen may also occur if the animal breathes through its mouth, as commonly occurs in dogs [42]. Flow‐by oxygen supplementation, which consists of placing an oxygen source within approximately 2 cm of a patient’s nostrils and mouth, is the easiest and simplest way of increasing FIO2 during emergency situations in small animals. Oxygen flow rates typically used in small animals with this technique range from 100–200 mL/kg/min and the resulting FIO2 has been reported to be 0.24 to 0.45 [42,43]. Unfortunately, the efficacy of this strategy is reduced in uncooperative patients as they typically move their heads away from the oxygen source. Oxygen supplementation via a face mask attached to an anesthesia breathing circuit is an effective means of increasing FIO2 in dogs, cats, neonatal foals, and calves. In many animals, sedation may be required for the animal to accept placement of a face mask. If a tight‐fitting face mask is attached to a small animal anesthesia breathing circuit delivering 100 mL/kg/min of oxygen, it may result in FIO2 levels > 0.8. In dogs, providing oxygen (100 mL/kg/min) through a tightly fitting face mask attached to a breathing circuit for 3 min prior to induction of anesthesia increased inspired oxygen concentration to 90%; while flow‐by oxygen supplementation at the same rate resulted in an inspired concentration of 30% [40]. The time to desaturation (defined as a pulse oximetry hemoglobin saturation of 90%) after propofol‐induced apnea was significantly longer in dogs receiving preoxygenation by a face mask (approximately 3 min [187 ± 67 s]) than in animals receiving flow‐by oxygen (approximately 1 min [66 ± 17 s]) [40], consistent with a previous publication assessing preoxygenation with a facemask in healthy dogs [39]. Oxygen supplementation by face mask before inhalant anesthesia is a practical means of reducing the risk of hypoxemia caused by injectable induction agents in patients with respiratory disease. Although commonly used before induction of general anesthesia in small animals, face masks may not be suitable for delivering oxygen for prolonged periods due to lack of patient tolerance and the need for personnel to hold the mask to the animal. Delivery of oxygen using a nasal catheter is suitable for long‐term oxygen supplementation. Animals with respiratory disease may have a nasal oxygen system in place prior to anesthesia and/or require oxygen supplementation using this system following anesthesia. A short nasal catheter, consisting of two 1 cm long prongs designed for people, has been used to deliver oxygen with some success in dogs; however, they are easily dislodged and only fit a small subset of animals. Longer nasopharyngeal catheters, generally constructed of soft flexible material, ideally with multiple fenestrations, can be placed further into the nasal cavity and are less likely to be dislodged. They have been shown to be effective in delivering oxygen in a variety of species [44–48]. To avoid patient discomfort during catheter placement, a local anesthetic jelly is applied to the nostrils, followed by instillation of a local anesthetic solution into the nasal cavity with the animal’s head extended upwards. Sedation may be required in uncooperative patients and additional care should be taken not to exceed the recommended maximum dose of local anesthetic to avoid toxic effects in cats or other small patients. Catheters can be placed unilaterally or bilaterally in the ventral nasal meatus and extended distally into the nasal cavity or nasopharynx. In most dogs, advancing the catheter to the level of the medial canthus of the eye places its tip within the nasal cavity, while advancing it further to the level of the vertical ramus of the mandible places the catheter within the nasopharynx. In conscious animals, delivering oxygen at a flow rate of 30–150 mL/kg/min through a nasal cannula can result in an FIO2 of 0.3 to 0.7 (depending on species) [44,45,48]. Higher flow rates, while improving PaO2, can result in low patient tolerance and nasal mucosal damage [45,48]. In dogs, gastric tympany secondary to aerophagia has also been reported with high flow rates [49]. The effects of progressively increasing oxygen flow rates (from 50 to 200 mL/kg/min) through unilateral or bilateral nasal catheters in dogs and neonatal foals are illustrated in Fig. 38.3 [44,45]. As oxygen flow rates are increased, there are progressive increases in FIO2 and PaO2, without significant changes in PaCO2, from values obtained while animals are breathing room air [44,45]. Oxygen supplementation with bilateral catheters may result in slightly higher FIO2 and PaO2 in comparison to a unilateral nasal catheter. Patient tolerance to higher oxygen flow rates has also been reported to improve when nasal catheters are placed bilaterally compared to unilaterally in dogs [44]. To minimize the risk of oxygen toxicity caused by prolonged exposure to high FIO2 levels and other adverse reactions (low patient tolerance, excessive drying/irritation of the nasal mucosa, and aerophagia/gastric distension), the oxygen flow rate should be adjusted to the minimum necessary to maintain an acceptable hemoglobin saturation/PaO2 level (e.g., saturation > 90% or PaO2 > 60 mmHg). If a nasal catheter fails to maintain arterial oxygenation with high oxygen flow rates (> 150–200 mL/kg/min), or if the work of breathing does not improve despite increased oxygen levels, escalation to other methods of oxygen supplementation (high‐flow nasal oxygen therapy) or invasive mechanical ventilation should be considered. In horses sedated with detomidine and butorphanol, a nasal catheter providing 15 L/min (approximately, 30 mL/kg/min) of oxygen for 3 min increased PaO2 immediately after induction of anesthesia to 83 ± 20 mmHg (mean ± standard deviation) in comparison to PaO2 measured at the same time point in horses not receiving oxygen supplementation (56 ± 12 mmHg) [50]. Although this procedure appears effective to ameliorate the decrease in PaO2 immediately after induction of anesthesia with an injectable anesthetic, oxygenation relative to horses that were not administered nasal oxygen was not different after 30 min of anesthesia, and to date, there is no evidence to support routine preanesthetic nasal oxygen administration in healthy horses. Oxygen insufflation into the nasal cavity or trachea via a nasotracheal or orotracheal tube is commonly used in horses during recovery from general anesthesia. Delivery of 15 L/min oxygen through a nasotracheal catheter has been shown to result in PaO2 values greater than 90 mmHg in laterally recumbent horses during anesthetic recovery [51]. Administration of oxygen using a percutaneous transtracheal system has been described in foals in respiratory distress, as well as in healthy standing horses, and therefore should be considered for use in equine patients with impaired oxygenation [47,52]. Commercially available oxygen cages and hoods constructed of clear acrylic or plexiglass are available for use in small animals. Some commercially available cage designs permit the control of oxygen levels, environmental temperature, and humidity within the cage and are very effective for long‐term oxygen supplementation in small patients. Both systems are suitable for use prior to or following anesthesia but not during periods where access to the patient is required. Helmets designed for pediatric humans have been used to increase FIO2 and deliver continuous positive airway pressure (CPAP) in dogs recovering from anesthesia [53]. Figure 38.3 Effects of oxygen supplementation through unilateral or bilateral catheters advanced to the level of the nasopharynx on FIO2, PaO2, and PaCO2, (mean ± standard deviation) in conscious adult dogs (A.) and in neonatal foals (B.). The FIO2 in both studies was measured by an intratracheal catheter connected to an airway gas analyzer. The gray shaded area shows the PaCO2 range considered normal for most species (35 to 45 mmHg). See text for further explanation. Source: Data compiled from Dunphy et al. [44]; Wong et al. [45]. In contrast to low‐flow oxygen therapy systems discussed above, where the maximum oxygen flow rate is limited to 15 L/min, high‐flow nasal oxygen therapy (HFNOT) systems can deliver up to 60 L/min of humidified and heated oxygen or oxygen‐enriched air. The system includes an oxygen cylinder (or oxygen concentrator), an air mixer, a humidifier, heated tubing of varying sizes, and purpose‐made nasal prongs [42,54]. While low‐flow systems result in variable effects on FIO2 because of variations in V̇E and peak inspiratory flow, HFNOT systems reliably achieve the target FIO2 level because the high flow delivered to the airway exceeds the patient’s peak inspiratory flow [55]. In addition to a more reliable increase in FIO2 than traditional oxygen supplementation systems, HFNOT systems create a low level of CPAP, which may increase the functional residual capacity (FRC) and improve matching between ventilation and perfusion within the lungs, while still permitting the patient to breathe spontaneously [56–58]. The CPAP associated with HFNOT may also stent the airways opened, decreasing the work of breathing and mitigating the need for invasive mechanical ventilation in some patients [56]. The relative humidity of inspired gas provided by HFNOT systems is close to 100% at a temperature between 34 and 37 °C, thereby reducing the sensation of respiratory distress or mouth dryness in humans [59]. Because inspired gas flow is heated and humified, HFNOT may also result in better patient tolerance by not desiccating the nasal mucosa [59]. Based on human recommendations, the soft silicone binasal prongs used for HFNOT should occlude up to 50% of the nares in veterinary patients [56,60,61]. Preliminary canine studies have shown that the ability of HFNOT to achieve the target FIO2 and CPAP is dependent on the flow rate of oxygen or oxygen‐enriched air delivered to the patient. Oxygen flow rates between 1 to 2 L/kg/min achieve FIO2 levels > 0.95 in dogs [56] (Fig. 38.4). Despite the advantages of HFNOT over traditional oxygen supplementation systems, there are some conditions that may limit the clinical utility of HFNOT systems in veterinary patients, including patient intolerance, and the need to adapt the bilateral nasal prongs made for pediatric and adult humans to a variety of head sizes and conformations. In very small patients, it has been suggested to apply only one nasal prong as placement of bilateral pediatric nasal prongs may occlude more than 50% of the nares and impair expiratory flow [62]. Another disadvantage is the decrease in FIO2 below the target level in animals that are open‐mouth breathing because the oxygen‐rich gas delivered by the HFNOT system is diluted with ambient air entering through the mouth. Although it is possible that the HFNOT systems are less affected by panting/open‐mouth breathing, this hypothesis has not yet been evaluated [42,54]. Although morbidity associated with HFNOT is considered minimal, aerophagia leading to gastric distension is a potential complication of this therapy in dogs and may require placement of an orogastric tube [56,62,63]. HFNOT has the potential for use during the perianesthetic period or when orotracheal intubation is not feasible in anesthetized/deeply sedated patients. Because of the flow‐dependent increase in pharyngeal pressures/CPAP reported in dogs receiving 100% oxygen at flow rates ≥ 1 L/kg/min [56], the use of HFNOT may be particularly useful to reduce the inspiratory work of breathing and alleviate signs of upper airway obstruction of dogs with brachycephalic obstructive airway syndrome (BOAS). In one case series report, application of HFNOT to brachycephalic dogs with clinical signs of upper airway obstruction (dyspnea/inspiratory stridor) during recovery from anesthesia improved the respiratory pattern (decreased inspiratory stridor) [62]. However, results were limited by the small number of animals (n = 5) and by the lack of another group not receiving HFNOT for comparison [54]. In another case series report, HFNOT set to deliver 1 L/kg/min of 100% oxygen (33 °C) was evaluated in non‐intubated dogs anesthetized with propofol for bronchoscopy [64]. Of the four animals enrolled in this study, two animals showed transient hypoxemia (pulse oximetry saturation of 84% and 92% lasting < 1 min) during or after completion of bronchoalveolar lavage [64]. Delivery of high‐flow nasal oxygen may not be enough to counteract hypoxemia secondary to bronchoalveolar lavage, but conclusions of this case series were also limited by the small number of animals and by the lack of a control treatment. Figure 38.4 Effects of oxygen supplementation on airway pressure and FIO2 (mean and lower‐upper range) measured at the level of the oropharynx in dogs using A. a traditional nasal cannula and B. a high‐flow nasal cannula. Treatments were administered in randomized crossover design and included non‐sedated patients (traditional nasal cannula) and sedated/non‐sedated animals (high‐flow nasal cannula). With the high‐flow nasal oxygen therapy (HFNOT) system, the heated and humidified oxygen source was selected to deliver an FIO2 of 1.0. Notice that low‐flow oxygen supplementation (traditional nasal cannula) does not provide continuous positive airway pressure (CPAP) and has variable effects on FIO2, while the HFNOT adjusted to deliver oxygen flow rates between 1 to 2 L/kg/min can produce low to moderate levels of continuous positive airway pressure (CPAP) levels and reliably results in FIO2 close to the target level (1.0). Increasing the oxygen flow rate above 2 L/kg/min did not induce additional changes in CPAP and FIO2 and increased patient intolerance. Source: Data compiled from Jagodich et al. [56]. HFNOT systems have also been evaluated in animals with hypoxemic respiratory failure, where the most frequent pathology was pneumonia/aspiration pneumonia [65–68]. Collective analysis of these studies shows that HFNOT should be considered if traditional oxygen supplementation methods fail to improve patient’s oxygenation or cannot mitigate the increased respiratory effort/work of breathing. Under these circumstances, HFNOT may be effective in improving PaO2 and reducing signs of dyspnea, thereby avoiding escalation to invasive mechanical ventilation in some cases. It has long been recognized that high inspired oxygen levels (over 70%) when administered for prolonged periods will produce pulmonary dysfunction and even death in previously healthy laboratory and domestic animals [69]. Studies in animals with experimentally induced lung injury have also demonstrated that lung injury is negatively impacted by high FIO2 [70–73]. The onset of pulmonary damage in healthy animals seems to require FIO2 > 0.7, producing PaO2 values exceeding 450 mmHg and “prolonged exposure,” generally longer than the period usually associated with clinical veterinary anesthesia. While high FIO2 may promote atelectasis, there is no evidence that the use of 100% oxygen as the carrier gas during anesthesia negatively impacts outcomes in clinical veterinary patients. In critically ill humans, current recommendations include avoiding supramaximal PaO2 and maintaining PaO2 and SaO2 within a physiologic range [74–77]. Therefore, in the critical care setting, if spontaneously breathing patients receive oxygen supplementation, or if escalation to invasive mechanical ventilation is necessary, the general approach is to adjust the FIO2 to the point where PaO2 levels are in the range of 60–80 mmHg with hemoglobin oxygen saturation levels between 90–94% [76]. With severe pulmonary parenchymal injury in humans, there is current discussion of targeting some degree of “permissive hypoxemia” [78–80]. To date, the impact of permissive hypoxemia on mortality has not been clearly demonstrated [80,81]. Mechanical ventilators can be described as flow generators, driving gas into the lungs by creating a pressure gradient between the airway opening (Pao, pressure measured at the distal end of the endotracheal tube as airway pressure) and the alveoli (Palv, alveolar pressure). Inspiration ceases after the peak airway pressure (Ppeak) or volume set on the ventilator is achieved and, once the expiratory valve is opened, the elastic recoil of the lungs and chest wall results in passive expiration. While intensive care unit (ICU) ventilators vent exhaled gases out into the environment, anesthesia ventilators are designed to allow rebreathing of expired gases. The primary goals of mechanical ventilation during anesthesia are to optimize gas exchange and reduce the work of breathing while minimizing the risk of iatrogenic lung injury. In addition to its beneficial effects on respiratory function, mechanical ventilation may facilitate the control of depth of inhalant anesthesia when compared to spontaneous breathing. In anesthetized, spontaneously breathing animals, changes in the respiratory pattern (respiratory rate [f] and tidal volume [VT]) leading to variations in minute ventilation (V̇E = f × VT) will modify the rate at which the alveolar (end‐tidal) anesthetic concentration approaches the inspired anesthetic concentration. As a practical example, if an animal hypoventilates significantly (decreased V̇E) immediately after induction of anesthesia, the decreased V̇E will slow down the rate of rise in end‐tidal anesthetic concentration, potentially delaying the time until an adequate depth of anesthesia is achieved. Alternatively, if the animal receives mechanical ventilation and the V̇E is adjusted to prevent hypoventilation, the end‐tidal anesthetic concentration will increase more rapidly. The first mention of mechanical ventilation in the form of positive‐pressure ventilation dates back to the mid‐1500s when the Dutch physician at the University of Padua (Italy) Andreas Vesalius observed that “… life may be restored to the animal, an opening must be attempted in the trunk of the trachea, into which a tube of reed or cane should be put; you will then blow into this, so that the lung may rise again and take air” [82]. Early mechanical ventilators were designed to establish a negative (sub‐atmospheric) pressure around the thorax to enlarge the chest wall and pull gas into the lungs by creating a negative intrapleural/alveolar pressure. After the development of rudimentary models of negative‐pressure ventilators between the second half of the 1800s and early 1900s, the first negative‐pressure ventilator effectively used for life support in humans with respiratory muscle paralysis was developed from studies in curare‐paralyzed cats by Philip Drinker and Louis Agassiz Shaw in the United States. These ventilators were named “iron lungs” and became widely used for life support in human patients suffering from respiratory muscle paralysis secondary to poliomyelitis during the 1950s [83]. Due to the required equipment and limited access to the patient’s thorax, abdomen, and limbs, ventilatory support using negative‐pressure ventilation cannot be applied to anesthesia machines designed for use in the operating room or the modern ICU setting. Instead, mechanical ventilation under these circumstances can be more effectively adopted by applying positive pressure to the airways. When William Thomas Green Morton performed the first official demonstration that surgery could be carried out without pain by administration of an inhalant anesthetic on October 16, 1846 (Ether Day), early inhalant anesthetic delivery systems were simple inhalers whose aim was to evaporate the anesthetic agent as a function of the patient’s own breath. This rudimentary equipment evolved during the first decades of the 20th century to anesthesia machines that allowed precise administration of an anesthetic gas flow independent of the patient’s breathing effort. Further developments included CO2 absorption and the circle breathing system, which allowed rebreathing of inhalant anesthetics and oxygen. However, it was only in the 1950s that anesthesia ventilators were effectively integrated into inhalant anesthetic delivery systems [84,85] (Fig. 38.5). Conventional anesthesia ventilators used in veterinary practice operate solely in a volumetric mode where the user adjusts the inspiratory flow and/or inspiratory time to achieve the target volume displaced by the bellows or, alternatively, the target Ppeak (Fig. 38.6A and B). As discussed further below, the volume displaced by the bellows may not accurately reflect the VT delivered to the animal [86]. There are two types of ventilators according to the movement of the bellows during expiration: ascending and descending bellows. Early ventilators were designed with bellows that descended during expiration (Fig. 38.5E and F). Unfortunately, in case of a leak in the circuit and/or accidental disconnection of the breathing circuit, the operator may be unaware of ventilator failure because a descending bellows will continue to move upwards during inspiration and downwards during expiration as gravity will cause the bellows to fill with ambient air. In contrast, ascending bellows ventilators have the advantage of providing an early warning in case of disconnection or a large leak in the breathing circuit because the bellows will not move upwards during expiration. Providing that the fresh gas flow (FGF) delivered by the flowmeter is not elevated, small leaks within the breathing circuit can also be detected by an ascending bellows as it will not completely fill/reach the top of its housing during expiration. Because there is a requirement for a compressed gas source to drive the ascending bellows downwards during inspiration and generate the tidal breath, these devices are classified as pneumatic ventilators. While older models did not require electricity, several bellows‐type ventilators used in veterinary medicine incorporate electronically controlled parameters (e.g., respiratory rate and inspiratory time or inspiration‐to‐expiration [I:E] ratio) and require both compressed gas and electricity to function (Fig. 38.6A and B). Ventilators designed for use in the magnetic resonance imaging environment require only compressed gas and do not have electronic components. The interval at which each mechanical breath is initiated is set by the respiratory rate control knob. Because termination of each mechanical breath occurs after a predetermined time has elapsed, which corresponds to the inspiratory time, these devices are classified as time‐cycled ventilators. In some electronically controlled bellows‐type ventilators, the inspiratory time is indirectly defined by adjusting the respiratory rate and the I:E ratio (Fig. 38.6A), whereas for other ventilators, there is a control knob to adjust the inspiratory time, and the I:E ratio is indirectly defined as a function of the inspiratory time and breathing frequency (Fig. 38.6B). Fig. 38.7 illustrates a circle breathing circuit attached to an ascending bellows‐type ventilator. These ventilators are also known as “double circuit ventilators,” meaning that the drive gas and the breathing gas circuit exist in two separate circuits. During inspiration (Fig. 38.7A), the driving gas (compressed air or oxygen) is routed into the bellows housing, which causes the bellows to move downwards. Airway pressure increases as the bellows is compressed, and the VT is delivered to the patient. The degree of compression of the bellows/VT delivered to the patient is set by adjusting the inspiratory flow knob until the volume displaced by the bellows (as shown by a volume scale located in the bellows housing) matches the target VT. However, the volume displaced by the bellows may be an inaccurate estimate of the actual VT delivered to the patient [86] as the inspired VT is not solely determined by the volume displaced by the bellows. Inspired VT will also be influenced by compliance and leaks within the breathing circuit and by the FGF. As an alternative to setting a predefined VT based on the volume displaced by the bellows, the inspiratory flow may be adjusted until Ppeak (assessed by the breathing circuit pressure gauge) increases to a certain level. Figure 38.5 Early models of anesthesia ventilators incorporated into anesthetic delivery systems. A. and B. The Dräger Romulus (Germany) and the Engstrom Model 150 (Sweden) were integrated with ventilators that applied positive pressure to the airways to expand the lungs during inspiration. During expiration, negative pressure was applied to the airways to “remove residual air from the lungs,” a concept that is no longer utilized. The ascending bellows design (classification based on the movement of the bellows during expiration) of the Dräger Pulmomat ventilator is still used by contemporary anesthesia ventilators. C. and D. The BOC Boyle Machine (United Kingdom) and the Foregger Eiffel Anesthesia Machines (USA) did not incorporate anesthesia ventilators and ventilation had to be performed by manually squeezing the reservoir bag. E. As mechanical ventilation became increasingly popular in human anesthesia in North America, the Bird Corporation developed a stand‐alone anesthesia ventilator that could be integrated into pre‐existing anesthesia machines by replacing the reservoir bag of the circle circuit with a descending bellows ventilator (Bird Mark 4). F. Ohio Medical Products began integrating anesthesia ventilators into their equipment in the 1960s. Source: Reproduced with permission from the Wood Library Museum of Anesthesiology. Delivery of pressurized drive gas into the bellows housing is interrupted during expiration. As passive expiration occurs through the elastic recoil of the lungs and chest wall, the bellows fills with expired gas and fresh gas entering the circuit, while the exhalation valve opens to allow the drive gas to be vented out to the room or the exterior/anesthetic gas absorber through the scavenge outlet (Fig. 38.7B). When the bellows is full, excess FGF will cause pressure build‐up within the breathing circuit. As pressure in the circuit reaches 2–4 cmH2O, the ventilator pop‐off valve (also known as the “spill valve”) opens and excess breathing circuit gas is vented out through the scavenge outlet (Fig. 38.7C). Because of the weight of the passive bellows and of the pressure necessary to open the ventilator pop‐off valve, ascending bellows ventilators typically result in an intrinsic positive end‐expiratory pressure (PEEP) of 2–4 cmH2O. In large animal anesthesia machines, the weight of the ascending bellows results in higher intrinsic pressures. During mechanical ventilation, part of the volume displaced by the bellows may get compressed within the breathing circuit before reaching the lungs. Gas compression losses cause the volume delivered to the lungs (inspired VT) to be less than the volume displaced by the bellows. The volume of compressed gas within one ventilator model was found to increase linearly at a ratio of 3.8 mL for every 1 cmH2O increase in airway pressure [88]. Also, part of the volume generated by the ventilator may not reach the patient because of the compliance of the breathing circuit. The latter is defined as the change in volume (distension of breathing circuit tubing) caused by an increase in pressure (mL/cmH2O). Breathing circuit compliance losses causing a decrease in inspired VT depend on the level of positive pressure achieved within the circuit, and on the diameter and length of the corrugated tubing. According to international standards, breathing circuit compliance losses should ideally be less than 1 mL per cm H2O of distending pressure for every 1 meter of tubing [89]. If that is the case and the anesthesia circuit for medium/large size dogs is 3 meters in total length for the inspiratory and expiratory limbs, mechanical ventilation to a Ppeak of 10 cmH2O will result in a delivered VT of 30 mL less than the volume displaced by the bellows. Besides compliance and gas compression losses, which may vary according to tubing characteristics and total internal volume of the breathing circuit, leaks within the breathing circuit are an important source of discrepancy between the volume delivered by the bellows and inspired VT. Therefore, to prevent accidents such as failure to properly ventilate an anesthetized animal, a thorough preanesthetic assessment of the ventilator, including a leak test of the breathing circuit, should be performed prior to use. Figure 38.6 Examples of time‐cycled, electronically controlled, ascending bellows ventilators designed for veterinary use that can be fitted into pre‐existing anesthesia machines. These ventilators operate only in volume‐control mode (mandatory breaths only), where the operator sets the breathing rate, I:E ratio (or inspiratory time), and the volume displaced by the bellows. Some ventilators incorporate a pressure limit control to prevent an excessive increase in peak airway pressure (Ppeak). The inspiratory flow control knob adjusts the flow of compressed gas (e.g., oxygen) that drives the bellows downwards, regulating volume that enters the breathing circuit. During small animal anesthesia, the inspired VT may differ substantially from the volume displaced by the bellows [86], therefore it may be preferable to perform pressure‐limited ventilation by adjusting the inspiratory flow to achieve a target Ppeak (based on the pressure gauge of the breathing circuit) instead of a target volume. In a conventional anesthesia apparatus, the portion of FGF entering the breathing circuit during inspiration adds to the volume delivered by the bellows to the lungs and increases Ppeak, an effect known as “fresh gas coupling” (Fig. 38.7A) [86,87,90,91]. Activation of the oxygen flush valve during inspiration, which delivers a high flow of 100% oxygen directly into the breathing circuit, will also generate excessively high Ppeak values and increase inspired VT if the apparatus does not incorporate an appropriately adjusted inspiratory pressure limiter (maximum airway pressure‐control) (Fig. 38.6A). If FGF is relatively low (e.g., ≤ 50 mL/kg/min), the added volume to the VT delivered by the bellows may not be clinically relevant; however, if FGF is high (relative to the size of the patient), it can substantially increase the VT. As shown in Table 38.1, in an anesthetized dog weighing 20 kg, where the ventilator is set to deliver 15 mL/kg based on the bellows scale (300 mL), with a respiratory rate of 10 breaths/min and an I:E ratio of 1:2 (2 s of inspiratory time), if the FGF is set at 1 L/min (50 mL/kg/min), approximately 16.6 mL of fresh gas will enter the breathing circuit every second. Therefore, during the inspiratory phase of each mechanical breath (2 s), a total volume of 33.3 mL is added to the volume of gas delivered by the bellows. When this added FGF volume is balanced against losses from breathing circuit compliance and gas compression, one may expect that the inspired VT will be close to 300 mL because the relatively small volume added by the FGF during inspiration (33.3 mL) will be close to breathing circuit compliance and gas compression losses (39 mL) (Table 38.1). If FGF is increased from 1 to 4 L/min, 66.6 mL of fresh gas will be entering the breathing circuit every second, which could potentially increase the inspired VT from 294 mL (14.7 mL/kg) to 379 mL (19 mL/kg) (Table 38.1). While fresh gas coupling may have a smaller impact on the VT delivered for medium‐sized/large dogs, this effect may be particularly hazardous during anesthesia of animals weighing ≤ 5 kg. If the FGF is increased from 1 to 4 L/min in a 3.3 kg cat, the inspired VT may increase by approximately 126%, from 48 mL (14.5 mL/kg) to 113 mL (34.2 mL/kg). Such supraphysiological VT, in addition to being harmful to the lungs, will result in elevated Ppeak and could result in greater cardiovascular depression due to decreased venous return and cardiac output. In contrast, the effects of FGF on the VT in a large animal anesthesia ventilator are not clinically relevant. Assuming a VT delivered by a large animal ventilator (based on the scale of the bellows housing) is 6 L for a 500 kg horse and the inspiratory time is set at 2 s, the additional volume generated by a 6 L/min FGF will represent less than 5% of the set VT (6 L plus 200 mL of FGF) and will likely be minimized by breathing circuit compliance and gas compression losses. As discussed below, modern anesthesia ventilators either decouple the FGF from the inspired VT or compensate for the effect of FGF by adjusting the inspired volume generated by the ventilator to achieve the user‐set VT [87,90,91]. When using a ventilator that does not decouple or compensate for changes in FGF, the user must exert caution when adjusting the FGF on the anesthetic machine. If FGF is increased, the circuit pressure should be monitored, and the inspiratory flow (tidal volume control) reduced to maintain Ppeak at a target value to avoid lung overdistention. As accurate measurement of inspired and expired VT (via spirometry) is not readily available in many veterinary anesthesia ventilators, the operator may adjust the inspiratory flow to achieve a target Ppeak (e.g., 8–10 cmH2O for medium‐sized dogs). Further adjustments in Ppeak and respiratory rate are performed with the aim of maintaining end‐tidal carbon dioxide partial pressure (PETCO2) within a predefined range (e.g., between 35 and 45 mmHg). Figure 38.7 Dynamics of gas flow in a breathing circuit of a conventional anesthesia apparatus equipped with a bellows‐type ventilator. See text for further details. Source: Adapted from Bokoch and Weston [87]. Figure 38.8 Modern anesthesia workstation (EX‐35Vet, Mindray Animal Care) incorporating an ascending bellows ventilator (1). Interchange between spontaneous and mechanical ventilation is performed by a switch (2). The pop‐off (adjustable pressure‐limiting [APL]) valve) (3) and the reservoir bag (4) remain active only during spontaneous ventilation. During mechanical ventilation, flow sensors placed close to the inspiratory (5) and expiratory (6) valves of the breathing circuit provide accurate monitoring of inspired VT and expired VT (7) and allow display of the inspiratory and expiratory flow curves over time (8). A pressure transducer within the breathing circuit monitors airway pressures during inspiration and expiration and allows the graphical display of airway pressure changes over time (9). Continuous monitoring of airway pressure and volume changes throughout the respiratory cycle provides the graphical display of pressure–volume (P–V) loops (10). Airway resistance (Raw) is calculated from the difference between plateau pressure (Pplat) and positive end‐expiratory pressure (PEEP) divided by the peak expiratory flow, while compliance (Compl) is calculated as the ratio between tidal volume (VT) and the driving pressure (Pplat − PEEP). Table 38.1 Effects of fresh gas flow (FGF), breathing circuit compliance, and gas compression on the inspired tidal volume (VT) in a 20 kg dog and in a 3.3 kg cat anesthetized with a bellows‐type ventilator where the volume delivered by the bellows was adjusted to 15 mL/kg. a Assuming a fixed inspiratory time (2 s): 10 breaths/min and I:E ratio = 1:2. b Assuming a compliance loss of 0.3 mL per 1 cmH2O of distending pressure for every 1 m of breathing circuit corrugated tubing (1.5 m inspiratory hose + 1.5 m expiratory hose = 3 m of tubing length). c Assuming a gas compression loss of 3 mL for every 1 cm H2O of distending pressure. d Inspired VT = Volume delivered by the bellows + (Volume added during inspiration by the FGF – [breathing circuit compliance losses + gas compression losses]). FGF, fresh gas flow; VT, tidal volume; Ppeak, peak airway pressure. Figure 38.9 Bland–Altmann plots showing percentage error of expired VT measured by proximal and distal flow sensors in relation to VTs generated by a pediatric lung model (ASL). A. Near‐patient spirometer (pediatric D‐Lyte, GE Healthcare) and B. Variable orifice inbuilt spirometer (Aisys CS2, GE Healthcare). The mean bias (continuous line) and precision (dashed lines) show better agreement and precision of the near‐patient spirometer with the VTs generated by the simulated lung model. Differences were most significant with VTs used in pediatric/neonatal humans or animals weighing ≤ 5 kg (VT ≤ 50 mL, red ovals), suggesting that near‐patient spirometry could be preferable under these circumstances. Source: Morgenroth et al. [98]; reproduced with permission. Modern anesthesia ventilators incorporate many features that were previously available only in ICU ventilators. Some ventilators incorporate pressure transducers that permit the accurate measurement of breathing circuit pressure and can display airway pressure‐time curves. Advanced anesthesia ventilators also incorporate flow sensors (spirometers) placed between the breathing circuit and the endotracheal tube (proximal or near‐patient spirometry) or placed near the inspiratory and expiratory valves (distal or inbuilt spirometry) of the breathing circuit and display flow‐time curves (Fig. 38.8). Numerical integration of the flow signal by the ventilator permits accurate monitoring of inspired and expired VT on a breath‐by‐breath basis. In addition to these variables, incorporation of flow sensors and airway pressure transducers to anesthesia ventilators provides real‐time monitoring of pressure–volume (P–V) loops, that is, the graphic display of changes in airway pressure (∆P) plotted as a function of changes in lung volume (∆V), and of changes in flow versus changes in lung volume (flow‐volume loops) [92]. These parameters, combined with arterial blood gases and other non‐invasive monitoring tools (capnography and pulse oximetry), provide the basis for adjusting ventilation parameters. For anesthesia ventilators that do not incorporate built‐in flow sensors, a multiparameter monitor that uses a near‐patient/proximal flow sensor (Pitot tube) originally designed for humans can be used to accurately monitor VT in small animals [92,93]. This same flow sensor refashioned to fit between a large animal endotracheal tube and the Y‐piece of a large animal anesthesia machine has been used to accurately monitor VT during mechanical ventilation in horses and other large animals [94,95]. Monitoring VT by means of proximal/near‐patient flow sensors more accurately reflects the actual VT delivered during mechanical ventilation in pediatric/neonatal humans than distal flow sensors placed near the expiratory valve [96,97]. For an ascending bellows human anesthesia ventilator (Aisys CS2, GE Healthcare), VT measurements provided by a proximal flow sensor also resulted in more accurate and precise estimates of VT than a variable orifice flow sensor placed near the inspiratory and expiratory valves of the breathing circuit (Fig. 38.9) [98]. Although proximal flow sensors have some disadvantages (e.g., inaccuracy in the presence of excess humidity) in comparison to distal flow sensors, their performance may be superior for neonatal/pediatric humans and for animals weighing ≤ 5 kg [98]. This difference in performance may be explained by different sensor technologies and the fact that sensors placed near the endotracheal tube may achieve better signal‐to‐noise ratio; that is, the level of the desired signal (flow) is higher in comparison to the level of background noise/interference (which causes inaccuracy). The reader is referred to the specialized literature for additional details regarding advantages and disadvantages of different flow sensors used in anesthesia ventilators [92,99]. As previously described, there are two types of ventilators according to the movement of the bellows during expiration (ascending and descending bellows). Modern anesthesia ventilators usually incorporate the ascending bellows design, which allows visualization of leaks within the breathing circuit as the bellows does not completely fill during expiration. One disadvantage of bellows ventilators is the consumption of higher amounts of compressed gas (air or oxygen compressed within a cylinder) [87,90,91]. One veterinary anesthesia ventilator uses a turbine to generate the compressed gas (ambient air) necessary for driving the ventilator bellows (Veta 5, Mindray Animal Health). A potential problem associated with ventilators that use compressed gas to drive the ventilator bellows is excessive VT delivery (and possibly barotrauma) if there is a communication due to a tear or leak between the bellows and the housing assembly because high‐pressure driving gas can enter the patient circuit. Inhalant anesthetic concentrations within the breathing circuit may be abnormally low as breathing circuit contamination by drive gas will lead to a dilutional effect. If the bellows is driven by compressed air, breathing circuit contamination by the drive gas will lower the inspired oxygen concentration [87,90,91]. The major advantage of modern anesthesia ventilators is that changes in FGF do not alter their ability to deliver an accurate VT. This is achieved by either the ventilator reducing the volume delivered to compensate for the FGF entering the circuit during inspiration (fresh gas flow compensation) or by preventing fresh gas from entering the breathing circuit during inspiration (fresh gas decoupling). Fresh gas flow compensation is used by bellows‐type ventilators, whereas fresh gas decoupling has been used by piston‐driven ventilators originally designed for human anesthesia [87,90,91]. Accuracy of inspired VT can also be guaranteed by compensation for losses caused by compliance of the breathing circuit with some ventilators [87,90,91]. Advanced anesthesia workstations perform a mandatory self‐test before the machine becomes fully operational. Among other safety checks, the pre‐use self‐test may quantify volume losses caused by compliance of the breathing circuit (mL/cmH2O) and by small leaks (Fig. 38.10). These measurements are considered when the electronically adjusted VT is delivered to the patient (breathing circuit compliance compensation). To guarantee optimal ventilator performance, the pre‐use self‐test should be repeated every time the hoses and other components of the breathing circuit are changed between anesthetic procedures and the flow sensors should be calibrated as per manufacturer recommendations. Fig. 38.11 illustrates the dynamics of gas flow of an advanced anesthesia workstation equipped with an ascending bellows‐type ventilator. During inspiration (Fig. 38.11A), pressurized gas enters the bellows housing, driving the bellows downwards to deliver the VT. The bellows contains a mixture of exhaled gas and fresh gas, that will add to the fresh gas entering the circuit (fresh gas inlet). The VT set by the user on the screen of the ventilator electronically controls the gas flow that enters the bellows housing and drives the bellows downward, using data integrated from inspiratory and expiratory flow sensors to deliver an accurate VT. A stable VT delivered by the ventilator is guaranteed by a feedback signal provided by the inspiratory flow sensor placed near the inspiratory valve. The inspired VT is not altered by the FGF because the volume delivered by the bellows is constantly adjusted to compensate for the volume added by the FGF, and as mentioned, for breathing circuit compliance losses identified during the mandatory pre‐use self‐test. The expired VT is measured by a flow sensor placed near the expiratory valve and data from inspiratory and expiratory flow sensors is used by some anesthesia ventilators to compensate for compliance losses (Fig. 38.11B). Because the inspired VT does not account for small leaks around the endotracheal tube or supraglottic device, assessment of ventilation should be based on expired VT. While the bellows moves toward the top of the housing during passive expiration, the pop‐off valve (spill or pressure relief valve) of the ventilator remains closed and only the drive gas exits through the exhalation valve to the scavenge system. Once the bellows is filled, pressure built up within the system opens the ventilator pop‐off valve and allows excess gas from the breathing circuit to exit to the scavenge system (not shown) [87,90,91]. Figure 38.10 Screenshot of the mandatory self‐test of the Dräger Primus Anesthesia Workstation with a pediatric circle system. After pressurizing the system to 30 cmH2O (pop‐off/APL valve set to 30 cm H2O), breathing circuit compliance was 0.2 mL/cmH2O and leak was 7 mL/min. The green light means that the parameter passed the self‐test according to manufacturer standards. The volume delivered by the piston‐driven ventilator, in addition to being decoupled from the fresh gas flow, will compensate for compliance losses to deliver an accurate VT. While the bellows‐type ventilator is the most common type of ventilator used in veterinary medicine, newer ventilator technologies are available in modern anesthesia workstations originally designed for humans, including piston, volume reflective, and turbine ventilators. The reader is referred to the literature for more details on these newer anesthesia workstations [87]. One piston‐driven ventilator has been specifically designed for use in large animals (Tafonius, Hallowell EMC) and is described in Chapter 6. An electrically driven piston ventilator (Fig. 38.12) functions as a single‐circuit ventilator as there is not a second circuit with compressed gas to drive the piston. Instead, a stepped electric motor controls the movement of a piston within a cylinder to deliver the inspired VT. This driving mechanism has the advantage of significantly reducing the use of compressed gas, but in the event of a power failure, it will depend on limited battery backup [90]. The inspired VT is accurately delivered because of: (1) the low compliance of the piston chamber and the accuracy of the stepped electric motor; (2) the volume displaced by the piston is increased to compensate for breathing circuit compliance losses assessed during the pre‐use self‐test (compliance compensation) (Fig. 38.10); and (3) the FGF is decoupled during inspiration (decoupling valve closed) (Fig. 38.12A) [87,90,91]. During inspiration, the piston delivers a mixture of exhaled and fresh gas, and the FGF entering the circuit is diverted to the reservoir bag, which remains active during mechanical ventilation. In contrast to a bellows ventilator, excess circuit gas is vented through the scavenge outlet during inspiration (Fig. 38.12A). Expired gases pass through the CO2 absorber and initially fill the reservoir bag. As the decoupling valve opens, a mixture of expired and fresh gas from the reservoir bag and FGF entering the circuit fills the ventilator as the piston moves downwards to its original position (Fig. 38.12B). Figure 38.11 Dynamics of gas flow during A. inspiration, and B. expiration in a breathing circuit of an advanced anesthesia apparatus equipped with a bellows‐type ventilator (Aysis anesthesia workstation, GE Healthcare). The bellows‐type ventilator is positioned distal to the expiratory valve of the breathing circuit. Notice that the reservoir bag and the APL (pop‐off) valve are isolated (inactive) during mechanical ventilation. After the bellows reaches the top of the housing during expiration, excess gas within the breathing circuit opens the ventilator pop‐off valve once the pressure exceeds 2 cmH2O and exits to the scavenge outlet (not shown). See text for further explanation. Source: Modified from Bokoch and Weston [87]. Unlike the bellows‐type ventilator where the movement of the bellows can be directly visualized through its transparent housing, the piston within the cylinder is usually concealed from view by the operator. With the ascending bellows ventilator, an accidental disconnection or a large leak within the breathing circuit can be rapidly detected as the bellows will not fill during expiration; while smaller leaks will cause the bellows to incompletely fill. A leak in a piston‐driven ventilator may be more difficult to detect. If a leak is present, during expiration the cylinder will fill with ambient air by activation of a negative‐pressure valve, diluting inspired gases with air, decreasing FIO2, and reducing anesthetic gas concentration within the circuit (possibly leading to patient awareness). Collapse of the reservoir bag, which contains a mixture of expired and fresh gas during mechanical ventilation, provides the user with an alert of a large leak/disconnection in the breathing circuit of a piston ventilator. Figure 38.12 Dynamics of gas flow during A. inspiration, and B. expiration in a modern anesthesia apparatus equipped with a piston ventilator (Fabius GS anesthesia system, Dräger). A piston‐type ventilator is located between the fresh gas inlet and the inspiratory valve. Notice that the rebreathing bag remains active during mechanical ventilation and excess gas exits the breathing circuit during inspiration. See text for further explanation. Source: Modified from Bokoch and Weston [87]. In continuous mandatory ventilation (CMV), also known as “controlled ventilation,” the ventilator delivers all mechanical breaths at predefined intervals according to the respiratory rate set by the operator. Continuous mandatory modes of ventilation are typically time cycled, that is termination of the inspiratory phase occurs after a certain time has elapsed. As explained above, for some ventilators, the inspiratory time is directly adjusted by the operator, while for other machines inspiratory time is indirectly adjusted via changes in the I:E ratio and respiratory rate. When a standard VCV mode is chosen, the operator selects the VT to be delivered (target variable), in addition to other variables (e.g., respiratory rate, I:E ratio or inspiratory time, and the PEEP level). In VCV mode, the VT is the independent variable as it will remain constant, whereas Ppeak is the dependent variable as it will change in case of modifications in respiratory system compliance (CRS) and airway resistance (Raw). The inspiratory flow, which is automatically adjusted by the ventilator to achieve the target VT, will remain constant throughout inspiration (square wave flow‐time curve). Because of the constant inspiratory flow pattern, airway pressure measured within the breathing circuit (that is equivalent to Pao) increases in a quasilinear fashion until the end of inspiration (Fig. 38.13A). The peak increase in airway pressure (Ppeak) recorded at the end of constant flow inspiration reflects the combined effects of airway resistance (Raw) and compliance of the respiratory system (CRS) on airway pressures [100–103]. Therefore, during conventional mechanical ventilation with a constant VT, a temporal increase in Ppeak suggests a decrease in CRS or an increase in Raw, and vice versa (Fig. 38.13B and C): In healthy lungs, it is assumed that the increase in airway pressure will rapidly equilibrate with Palv, expanding alveoli across the lung tissue. However, in VCV mode without an inspiratory pause, the absence of a plateau pressure (Pplat) at the end of inspiration could favor an unequal distribution of inspiratory flow, leading to preferential volume delivery to alveoli with faster‐filling rates (Fig. 38.14). Alternatively, VCV may allow maintenance of a Pplat during inspiration [100–103]. This goal can be achieved by adding an inspiratory pause (period of zero flow) after the target VT is achieved, which is usually defined by the operator as percent of total inspiratory time. Maintenance of a Pplat may theoretically allow a more homogenous distribution of gas flow across the lung (Fig. 38.14). A pause in inspiratory flow during VCV allows measurement of Ppeak at the end of constant inspiratory flow (when the target VT is delivered) and of Pplat at the end of the inspiratory pause (Fig. 38.15A). The Pplat (and the driving pressure, calculated as the gradient between Pplat and the PEEP level) is influenced by VT and by CRS. Therefore, during mechanical ventilation with a constant VT, an increase in Pplat or driving pressure over time is indicative of a decrease in CRS (Fig. 38.15B): Figure 38.13 Diagrammatic representation of changes in airway pressure, inspiratory and expiratory flow, and lung volume during volume‐controlled ventilation without an inspiratory pause: A. in a normal patient, B. in a patient with decreased CRS, and C. in a patient with increased Raw. A decrease in CRS or an increase in Raw will increase Ppeak while VT remains unaltered. During passive expiration, a decrease in CRS (or an increase in its reciprocal elastance) accelerates lung emptying as the time until expiratory flow returns to zero and the time until lung volume returns to its resting position (FRC) is shortened. Otherwise, increased Raw slows lung emptying and the flow‐time curve takes longer to reach zero while lung volume takes longer to return to FRC. VT, tidal volume; CRS, respiratory system compliance; Raw, airway resistance; Ppeak, peak airway pressure; PEEP, positive end‐expired pressure; FRC, functional residual capacity. Source: Partially modified from Kaczka et al. [104]. Figure 38.14 Diagrammatic representation of distribution of inspiratory gas flow during volume‐controlled ventilation (VCV) in alveoli with different filling rates: (A) small airways with normal resistance to flow leading to alveoli with normal compliance (“fast” alveoli); (B) small airways with high resistance to flow (“slow” alveoli); and (C) alveoli with low compliance. In the case of constant inspiratory flow leading to a quasilinear increase in airway pressure (i.e., positive‐pressure ventilation without a plateau pressure), gas flow is preferentially distributed to ‘fast’ alveoli, which may be overdistended. Maintenance of a plateau pressure during inspiration theoretically favors homogeneous gas distribution to alveoli with different filling rates. Figure 38.15 Diagrammatic representation of changes in airway pressure, inspiratory and expiratory flow, and lung volume during volume‐controlled ventilation with an inspiratory pause: A. in a normal patient, B. in a patient with decreased CRS and C. in a patient with increased Raw. Setting an inspiratory pause during VCV may allow the differentiation of the effects of changes in CRS and Raw on pulmonary mechanics. A decrease in CRS (or an increase in its reciprocal, elastance) results in an increase in Pplat and driving pressure (Pplat – PEEP), without altering the VT and gradient between Ppeak and Pplat. An increase in Raw will increase the gradient between Ppeak and Pplat. Duration of expiratory flow and time for lung volume to return to FRC follow the pattern explained in Fig. 38.13. VT, tidal volume; CRS, respiratory system compliance; Raw, airway resistance; Ppeak, peak airway pressure; Pplat, plateau pressure; PEEP, positive end‐expired pressure; FRC, functional residual capacity. Source: Partially modified from Kaczka et al. [104]. As explained before, the Ppeak recorded in VCV mode is the distending pressure of the lungs that is necessary to overcome the resistive and viscoelastic properties of the respiratory system (Raw and CRS, respectively). In humans, the Pplat recorded at the end of an inspiratory pause (period of zero flow) of at least 0.5 s, may reflect the distending pressure of the lungs that is necessary to overcome viscoelastic properties of the respiratory system (CRS) per se, without the confounding effects of Raw [100,103]. Analysis of the airway pressure‐time curve and the gradient between Ppeak and Pplat during VCV with an inspiratory pause of at least 0.5 s may allow the discrimination of the effects of CRS and Raw on airway pressures [100,103] (Fig. 38.15B and C). Animals may have low CRS because of obesity, increased intra‐abdominal volume caused by pregnancy, distended abdominal viscera, pneumoperitoneum, shape of the chest wall (dogs with barrel‐shaped chest conformation), atelectasis, and pleural/parenchymal lung disease. As explained before, during ventilation in volume‐controlled mode, VT will remain constant while airway pressure increases or decreases as a function of changes in CRS. If VT and respiratory frequency are adjusted appropriately, V̇E and normal PaCO2 levels can be maintained despite decreases in CRS related to patient positioning or the surgical procedure (e.g., pneumoperitoneum) [101,105]. Since a decrease in CRS will increase airway pressure during constant volume ventilation, modern anesthesia machines allow adjusting the maximum airway pressure in VCV mode, that is, inspiratory flow will be interrupted before preset VT is achieved if the airway pressure limit set by the operator is exceeded. Airway resistance may also increase because of anesthetic equipment (e.g., relatively narrow/kinked endotracheal tube, narrow tubing of the breathing circuit) or because of pathophysiological changes in the airways (e.g., brachycephalic syndrome in dogs, tracheal stenosis, and partial obstruction of the airways by thick/viscous secretions or foreign material). The use of an inspiratory pause during VCV may be advantageous because it may allow discrimination of changes in pulmonary mechanics caused by increased Raw from those caused by decreased CRS (Fig. 38.16). The PCV mode is characterized by a decelerating flow‐time curve during inspiration. Unlike the VCV mode, the operator selects the maximum inspiratory pressure (Pmax) rather than the VT to be delivered to the patient. During PCV, the Pmax is the independent variable while VT is the dependent variable. Specifically, the Pmax set by the operator will remain constant while VT will vary as a function of changes in CRS and Raw. Therefore, if CRS decreases or Raw increases, inspiratory airflow and VT will decrease, and vice versa (Fig. 38.17) [104,106]. During inspiration, following a rapid rise in airway pressure, which coincides with the peak inspiratory flow, pressure increases in a more curvilinear fashion until the target airway pressure (Pmax) is achieved. The decelerating flow may reach zero before the expiratory valve opens and passive expiration begins creating an inspiratory pause/Pplat (Fig. 38.17). Because the ventilator generates a decelerating inspiratory flow, PCV does not result in a peak rise in airway pressure (Ppeak) that is observed at the end of a constant inspiratory flow (VCV mode). For some anesthesia ventilators the rise time (Tslope), that is the time elapsed for airway pressure to increase from PEEP to Pmax in PCV mode, can be adjusted. An increase in Tslope in relation to the total inspiratory time will lower the peak increase in inspiratory flow and shorten the period of zero flow during inspiration, and vice versa (Fig. 38.18). When using PCV, the anesthesiologist must be aware that changes in chest wall compliance associated with specific surgical conditions, such as laparoscopic surgery associated pneumoperitoneum, could decrease CRS and lead to some degree of alveolar collapse/hypoventilation because VT will fall [105]. Therefore, if there is a decrease in expired VT monitored by the flow sensor during PCV, the operator may need to reset airway pressure to a higher value to maintain VT and prevent hypercapnia. VCV has the advantage of maintaining a constant VT and V̇E; however, it may result in high airway pressures. Conversely, PCV does not guarantee that VT and V̇E are maintained but has the advantage of reducing alveolar stress by limiting the maximum airway pressure delivered to the lung [106]. To overcome the disadvantages of decreased VT and V̇E in face of decreased CRS and increased Raw during PCV, some anesthesia ventilators incorporate the option to perform a volume‐targeted (or volume‐guaranteed) PCV mode. This ventilation mode, also known as “dual‐mode ventilation,” combines the advantages of VCV and PCV (delivery of a fixed VT and decelerating inspiratory flow resulting in lower airway pressures). In volume‐targeted PCV, the user selects the VT while the ventilator adjusts the lowest possible airway pressure to achieve the preset volume [101,107]. In healthy dogs undergoing abdominal surgery (ovariohysterectomy), PCV targeting a VT of 10 mL/kg improved airway dynamics (lower Ppeak) and resulted in higher CRS but without a detectable improvement in gas exchange (PaCO2, PaO2, and alveolar‐to‐arterial oxygen gradient P(A‐a)O2 in comparison to VCV (VT = 10 mL/kg) during the intraoperative period [108]. Recent meta‐analysis studies in humans have shown that volume‐guaranteed PCV improves airway dynamics (lower Ppeak and Pplat with higher CRS) during two‐lung ventilation in comparison with VCV, while an effective improvement in PaO2 was detected only during one‐lung ventilation (OLV) [109]. Although these findings might apply to animals without lung disease, these results may not be reproduced in animals with lung disease. A summary of the differences between controlled ventilation modes (VCV, PCV, and volume‐guaranteed PCV) is presented in Table 38.2. Figure 38.16 Screenshots of an anesthesia ventilator (Dräger Fabius Plus, Drägerwerk AG & Co.) used for a 3.8 kg cat anesthetized with isoflurane during volume‐controlled ventilation. The ventilator was adjusted to obtain an expired VT of approximately 40 mL (10–11 mL/kg) with an inspiratory pause of 40% of inspiratory time. The respiratory rate was set to maintain PETCO2 close to 35–40 mmHg. A. After a 3 mm internal diameter endotracheal tube was placed, the airway pressure tracing is suggestive of increased Raw and there is an increased gradient between Ppeak and Pplat. B. Immediately after the 3 mm tube was replaced by a 4 mm inner diameter endotracheal tube, the gradient between Ppeak and Pplat decreased, denoting a decrease in Raw. PETCO2, end‐tidal carbon dioxide partial pressure; Ppeak, peak airway pressure; Pplat, plateau pressure; Raw, airway resistance; VT, expired tidal volume. Source: Drägerwerk AG & Co. Figure 38.17 Diagrammatic representation of changes in airway pressure, inspiratory and expiratory flow, and lung volume during pressure‐controlled ventilation: A. in a normal patient, B. in a patient with decreased CRS, and C. in a patient with increased Raw. Notice that as inspiratory flow decelerates, airway pressure increases in a more curvilinear fashion compared to volume‐controlled ventilation and there is no Ppeak. Because airway pressure set by the user is kept constant, inspiratory/expiratory flow and consequently VT will change as a function of changes in CRS and Raw. In a more rigid lung (decreased CRS), inspiratory/expiratory flow is decreased and more quickly returns to zero. The decrease in airflow results in decreased VT. If Raw is increased, inspiratory/expiratory flow also decreases, resulting in a decrease in VT. VT, tidal volume; CRS, respiratory system compliance; Raw, airway resistance; Pmax, maximum inspiratory pressure; PEEP, positive end‐expired pressure; FRC, functional residual capacity. Source: Partially modified from Kaczka et al. [104]. Assisted and assisted‐controlled ventilation modes may be available in some anesthesia ventilators. In the assisted mode, the ventilator detects a trigger signal from the patient, that is, the commencement of a spontaneous breathing effort, to provide a synchronized mechanical breath. The trigger signal of anesthesia ventilators is usually based on a pneumatic signal, that is, the inspiratory flow or on the level of negative airway pressure generated by a spontaneous breath. Detection of chest wall motion and diaphragmatic electromyograms (neurally adjusted ventilatory assist) are other forms of triggering assisted mechanical breaths adopted in ICU ventilators that may improve patient‐ventilator synchrony, and the reader is referred to the literature for detailed descriptions of these technologies [110,111]. Pressure support ventilation (PSV) is a modality of assisted ventilation where the ventilator delivers a target inspiratory pressure with a decelerating flow pattern when a spontaneous breathing effort is detected [101]. Delivery of an assisted breath is based on the level of negative airway pressure or the inspiratory flow generated by a spontaneous breathing effort. The operator can increase the sensitivity of the triggering mechanism either by decreasing the inspiratory flow or by adjusting the level of negative airway pressure to a less negative value, and vice versa. During PSV mode, delivery of mechanical breaths is dependent on the degree of central ventilatory drive. Therefore, depending on the suppression of central nervous system (CNS) sensitivity to CO2 and the level of relaxation of respiratory muscles by anesthetic drugs, the number of patient‐triggered mechanical breaths will vary and result in varying degrees of decreased V̇E/hypercapnia or may even result in apnea. Ventilators often offer PSV with safety backup ventilation to provide the patient with mandatory ventilation in case of complete suppression of the CNS ventilatory drive or excessive respiratory muscle relaxation/paralysis [101]. This mode of ventilation can be adopted for weaning the animal from the ventilator when there is enough ventilatory drive for the patient to initiate spontaneous breaths. Figure 38.18 Airway pressure and flow curves recorded during pressure‐controlled ventilation in a 10 kg dog (9 kg ideal body weight) anesthetized with isoflurane and a constant rate infusion of fentanyl. The Pmax was adjusted to 12 cmH2O aiming to deliver a VT of approximately 12 mL/kg (108 mL based on ideal body weight) at a respiratory frequency of 12 breaths/min and an I:E ratio of 1:2, which resulted in an inspiratory time of 1.7 s. A. Notice that when the rise time (Tslope) was adjusted to match the inspiratory time (1.7 s), there was no period of zero flow and the pressure rose in a curvilinear fashion until reaching Pmax. The measured Ppeak (Pmax) cannot be considered equivalent to the Ppeak measured in VCV mode because it was measured at the end of the period of decelerating flow and not at the end of a period of constant inspiratory flow that is typical of VCV. The measured Pplat also cannot be considered reliable because there is no period of zero flow before passive expiration begins. B. Notice that when the Tslope is adjusted to 0.6 s (approximately, 35% of inspiratory time), a period of zero flow is created before passive expiration begins. This results in the formation of a plateau in the airway pressure curve, which allows correct measurement of Pplat. Pmax, maximum inspiratory pressure; VT, tidal volume; Pplat, plateau pressure. Table 38.2 Summary characteristics of volume‐controlled ventilation (VCV), pressure‐controlled ventilation (PCV), and dual‐controlled (volume‐guaranteed PCV) ventilation. The target pressure set in PCV mode is better defined as Pmax as true Ppeak (as measured at the end of constant inspiratory flow in VCV mode) cannot be measured with the decelerating flow that is characteristic of PCV. VCV, volume‐controlled ventilation; PCV, pressure‐controlled ventilation; VT, tidal volume; V̇E, minute ventilation; CRS, respiratory system compliance; Raw, airway resistance; Ppeak, peak airway pressure; Pplat, plateau pressure. Another fundamental difference between controlled ventilation modes (VCV, PCV, and volume‐guaranteed PCV) and PSV is the mechanism of termination of inspiratory flow (also known as “cycling mechanism”) [112,113]. As explained before, termination of inspiratory flow, that is the transition between inspiration and expiration, in controlled ventilation modes occurs after a certain time has elapsed (inspiratory time), as defined by the operator. Cycling (that is passive exhalation) in PSV mode occurs after the inspiratory flow decelerates to a certain percentage of its peak. Typically, the end of inspiration can be set at 25% of peak inspiratory flow to avoid breath holding (period of zero flow during inspiration), which may be uncomfortable to a patient that is spontaneously breathing. Although patient‐ventilator asynchrony is avoided during PSV as the patient initiates the mechanical breaths, asynchrony may still occur if the duration of inspiratory flow exceeds the time of an active exhalation effort, a phenomenon known as “delayed cycling.” If the patient tries to exhale while positive airway pressure is still being applied to the airway, the major consequence of delayed cycling is the creation of PEEP, which may also impair the triggering of assisted breaths. Avoidance of patient‐ventilator asynchrony caused by delayed cycling can be achieved by shortening the inspiratory flow by increasing the percent of peak inspiratory flow at which the ventilator cycles to exhalation (Fig. 38.19A and B). Adjusting the flow‐cycling is of great relevance in ICU patients that are on PSV and the reader is referred to the literature for further details [112,113]. Figure 38.19 Changes in inspiratory and expiratory flow and airway pressure during pressure support ventilation (PSV). A. The oval shows an abnormal deflection in the airway pressure curve (additional increase in airway pressure) caused by active exhalation while the ventilator is still generating inspiratory flow. B. Lung model showing flow‐cycling (end of inspiration) at 10%, 25%, and 50% of peak inspiratory flow. Notice that when the percent of peak flow is increased, there is a shortening of inspiratory flow, which may avoid patient‐ventilator asynchrony caused by delayed cycling. Source: Hess [112]; Gentile [113], reproduced with permission. Spontaneous intermittent mandatory ventilation (SIMV) is an assisted‐controlled ventilation modality provided by some anesthesia ventilators. This ventilation mode allows mandatory mechanical breaths as selected by the operator (controlled ventilation) and patient‐triggered mechanical breaths (assisted ventilation), with the mandatory breaths either in volume‐control (SIMV‐VCV) or pressure‐control (SIMV‐PCV) mode [101]. During the expiratory phase following a mandatory breath, there is a trigger window at late expiration (set by the operator as percentage of expiratory time) where the ventilator algorithm responds to a pneumatic signal (negative airway pressure or inspiratory flow generated by the patient) with assisted mechanical breaths either in volume‐control (constant flow and volume targeted) or pressure‐control (decelerating flow and pressure‐targeted) mode. A pressure‐targeted flow cycled breath (PSV mode) is delivered if the patient makes spontaneous breathing efforts at any time during expiration outside the triggering window. If the patient has an increased ventilatory drive and assisted mechanical breaths superimpose on the time interval programmed by the ventilator to deliver a mandatory breath, pre‐programmed breaths are canceled, avoiding patient‐ventilator asynchrony (Fig. 38.20). Anesthesia ventilators with SIMV capabilities often present the flow and pressure curves originating from patient‐triggered breaths with a distinctive color, or another graphic hallmark, to allow the operator to discriminate patient‐triggered from mandatory breaths. The SIMV mode (either in pressure‐ or volume‐control mode) can be selected for maintenance of general anesthesia; absence of patient‐triggered breaths during anesthesia can be achieved by setting a proper V̇E (based on f and VT during SIMV‐VCV or f and Pmax during SIMV‐PCV) to maintain eucapnia and by ensuring an adequate depth of anesthesia. Alternatively, spontaneous breathing efforts can be abolished by administering a neuromuscular blocking agent during anesthesia. The SIMV mode can be useful as an alternative to controlled modes of ventilation (VCV and PCV) in animals with spontaneous breathing efforts despite proper V̇E and adequate depth of anesthesia (Fig 38.20). At the end of anesthesia, weaning the animal off the ventilator can be achieved by reducing the number of mandatory breaths to allow CO2 to build‐up and increase central ventilatory drive; once ventilatory drive is judged to be adequate, as observed by the number of patient‐triggered breaths, the ventilation mode can be switched to assisted (PSV), before allowing the patient to resume spontaneous ventilation. Dynamic P–V loops, which graphically present changes in airway pressure (∆P) on the x‐axis and changes in lung volume (∆V) on the y‐axis during a mechanical breath, provide important information regarding Raw and CRS. A summary of changes in dynamic P–V loops that may occur during VCV and PCV are illustrated in Fig. 38.21. Analysis of dynamic P–V loops reveals that lung volume during inspiration is less than lung volume recorded at the same airway pressure during expiration. The difference in lung volume during inflation and deflation occurs because some of the energy spent to distend the lungs is lost during deflation. The dissipating energy during lung inflation/deflation (defined by the area within the P–V loop), named “hysteresis,” is attributed to alveolar recruitment during inspiration and de‐recruitment during expiration, alveolar surface tension/surfactant, gas absorption, and stress relaxation of lung tissue [114,115]. Figure 38.20 Mechanical ventilation with SIMV‐VCV (9100c nxt, GE Healthcare) in a 15 kg dog with ventilator asynchrony in volume‐controlled ventilation (VCV) mode, despite an adequate depth of anesthesia and a PETCO2 between 32–34 mmHg (pulse oximetry = 98% with an FIO2 = 0.35). A. Ventilation mode was switched to SIMV‐VCV (operator settings shown). B. The airway pressure and flow curves of mandatory volume‐targeted breaths are displayed in yellow and green, respectively, while patient‐triggered breaths are displayed in blue. Patient‐triggered, volume‐targeted breaths (blue), are delivered only during the trigger window after a mandatory breath (window set at 50% of expiratory time); spontaneous breathing efforts outside the trigger window initiate pressure‐targeted breaths (PSV). Notice that if patient‐triggered breaths coincide with the timing of mandatory breaths, the mandatory cycle is canceled to avoid asynchrony (overlapping between assisted and controlled breaths). Standard veterinary anesthesia ventilators or older model human anesthesia workstations adapted for veterinary use usually can perform mechanical ventilation only in volume‐controlled mode, without the option for adding an inspiratory pause. The user sets the inspiratory flow necessary to achieve a target VT. Tidal volume monitoring may be restricted to the visual analysis of the volume displaced on the ventilator bellows during inspiration, which strays from the actual inspired VT [86], while airway pressure is monitored by a pressure gauge incorporated into the breathing circuit. If the anesthesia apparatus does not incorporate flow sensors and pressure transducers, pulmonary mechanics can be monitored in small and large animal species by a near‐patient spirometer (Pitot tube‐based flow sensor), originally designed for use during mechanical ventilation in humans [93–95]. The original flow sensor (adult and pediatric D‐lite) can be used in small animals or other species with a maximum VT < 2 L; whereas the sensor remodeled into a larger scale (H‐lite) can be used during anesthesia in large animals (e.g., horses). The sensor, placed between the endotracheal tube and the Y‐piece of the breathing circuit, provides real‐time monitoring of inspired and expired VT, inspired and expired minute volume, Ppeak, Pplat, PEEP, CRS, and P–V loops (Fig. 38.22). Figure 38.21 Diagrammatic representation of dynamic P–V loops recorded during volume‐controlled ventilation (VCV) and pressure‐controlled ventilation (PCV) in dogs. A. Reference pressure–volume (P–V) loop during VCV. The inspiratory limb is a near‐straight line because airway pressure increases in a quasilinear fashion with lung volume during VCV. The slope is represented by the red dotted line. B. An increase in Raw during VCV may increase the area of the expiratory limb (hysteresis) due to an increase in resistive expiratory work. The slope also decreases during VCV because Ppeak increases to compensate for the increase in Raw. C. A decrease in CRS during VCV decreases the slope of the P–V loop while hysteresis may not be significantly altered. D. Reference loop during PCV. The inspiratory limb has a more rounded shape compared to VCV because of the decelerating inspiratory flow. E. An increase in Raw during PCV increases hysteresis during inspiration and expiration (box‐shaped P–V loop) while the slope may be unaltered. F. A decrease in CRS decreases the slope of the P–V loop during PCV. VT, tidal volume; CRS, respiratory system compliance; Raw, airway resistance; Ppeak, peak airway pressure. Dynamic CRS can be measured during VCV as the ratio between the change in lung volume (VT) and the change in airway pressure (difference between Ppeak and PEEP): The Ppeak generated by a constant inspiratory flow during VCV is the pressure necessary to overcome the resistive and viscoelastic properties of the respiratory system [100–102]. Therefore, decreased dynamic CRS is not only attributed to factors that decrease lung (atelectasis and pulmonary edema) and chest wall (increased intra‐abdominal pressure and obesity) compliance, but also to factors that increase Raw (narrow/kinked endotracheal tube, viscous secretions in the airways, and bronchoconstriction) [100–102]. Static CRS, measured as the ratio between VT and the driving pressure (Pplat – PEEP) during conditions of zero flow, allows evaluation of the viscoelastic properties of the respiratory system per se, without the confounding effects of the frictional component of the work done (Raw) [100–102]. In humans, a Pplat created by the inspiratory hold (period of zero flow at end‐inspiration) should last for at least 2–5 s to allow measurement of true Palv after gas flow distribution between alveoli with faster and slower filling rates (Fig. 38.14) [100,103]. If the duration of the inspiratory pause is between 0.5 and 2 s, it may still mostly overcome the frictional resistance imposed by the airways, but such compliance measurements are better defined as quasistatic [100,103]. The algorithm used to calculate CRS may significantly impact evaluation of pulmonary mechanics. During VCV without an inspiratory pause, compliance measurements provided by the ventilator should be considered dynamic. If there is an inspiratory pause during VCV and the manufacturer’s algorithm calculates CRS from Pplat, compliance measurements can be considered quasistatic or static, depending on the duration of the inspiratory pause (Fig. 38.23). Close hemodynamic monitoring should be performed, especially if longer periods of inspiratory hold are performed (> 25% of inspiratory time). Maintaining alveolar distension for longer periods could have negative hemodynamic consequences attributed to longer‐lasting decreases in vena caval blood flow and stroke volume (due to increased Ppl during mechanical ventilation) and to more prolonged increases in right ventricular (RV) afterload secondary to compression of pulmonary capillaries in West zones I and II during the inspiratory hold [116]. Figure 38.22 A. Pitot tube‐based spirometer/flow sensor designed for human anesthesia that is compatible for use in small animals (D‐lite, GE/Datex‐Ohmeda) and the same sensor remodeled into a larger scale for use in large animals (H‐lite). B. Pressure–volume (P–V) loops measured by the D‐lite sensor in a 26 kg dog undergoing laparoscopic ovariectomy under pneumoperitoneum (intra‐abdominal pressure of 15 mmHg). Compared to the P–V loop recorded before abdominal insufflation (L1), the decreased slope observed after induction of pneumoperitoneum (L2), suggests worsening (decreased) dynamic CRS. C. Pressure–volume loops measured by the H‐lite sensor in a 400 kg horse undergoing colic surgery in dorsal recumbency. Compared to the loop recorded before the abdominal cavity was opened (white arrow), the increased slope observed after abdominal decompression (black arrow) suggests an improvement (increase) in dynamic CRS. For the H‐Lite actual tidal volumes (TV) and dynamic compliance (C) are obtained by multiplying the displayed values by 5. Source: Reproduced from Calice et al. [93]; Moens et al. [[94]], with permission from Elsevier. Compliance measurements usually are not indexed to body weight in adult humans. However, because of the large variation in body mass and size of the lungs among veterinary species, CRS should be indexed to body weight (mL/cmH2O/kg). In dorsally recumbent adult horses undergoing VCV with zero end‐expiratory pressure (FIO2 > 90%), dynamic CRS may be close to 0.4 mL/cmH2O/kg, which is considerably less than CRS in anesthetized dogs (FIO2 = 50%) during VCV and with 2–6 cmH2O PEEP (1.3 mL/cmH2O/kg) [117,118]. Poor CRS typically found during mechanical ventilation in large animal species is caused by impaired lung and chest wall compliance. Compression atelectasis associated with relaxation and cranial displacement of the diaphragm is a major contributing factor to the decrease in lung compliance observed in horses [1,119,120]. Factors such as increased intra‐abdominal pressure, pregnancy, and obesity may further aggravate compression atelectasis and lead to greater decreases in CRS. Factors found to influence CRS in mechanically ventilated dogs include body weight, body condition score, and shape of the chest wall [118,121]. Three types of chest wall conformation are recognized in dogs: barrel‐chested (wide chest at the sternum), deep‐chested (narrow chest at the sternum), and intermediate‐chested (chest area at sternum between the deep and barrel‐shaped chest). In dogs with a barrel‐shaped thorax, CRS may be reduced by approximately 20% in comparison to dogs with intermediate chest wall shape. Similar decreases in CRS may be observed in dogs that are overweight (high body condition score) in comparison to dogs with normal body condition scores (Fig. 38.24) [118]. Development of atelectasis during anesthesia is associated with oxygenation impairment and decreased lung compliance. Conventional mechanical ventilation, which often improves V̇E and prevents hypercapnia, may fail to reopen atelectatic lung areas and increase PaO2 levels. If poor lung compliance (decreased CRS) and low PaO2 values in relation to the FIO2 (PaO2/FIO2 ratio < 300 mmHg) are observed, a temporary increase in airway pressures aiming to reopen atelectatic lung areas, that is, an alveolar recruitment maneuver (ARM), may be considered to increase CRS and PaO2 levels. In the open‐lung approach, originally developed for clinical use in humans with acute respiratory distress syndrome (ARDS) during mechanical ventilation, collapsed alveoli are opened by temporary application of elevated inspiratory pressures and post‐recruitment PEEP is applied to prevent recurrence of atelectasis [122]. In addition to an improvement in pulmonary mechanics and gas exchange, preventing cyclic alveolar collapse reduces the progression of lung injury associated with mechanical ventilation in ARDS patients because of improved homogeneity in alveolar distention throughout the lung parenchyma [123,124]. In addition to their use in humans with ARDS in the ICU setting, ARMs are an effective means to increase CRS and PaO2 levels in anesthetized humans not suffering from ARDS, or those undergoing cardiac and thoracic surgeries [125]. Systematic reviews in humans have recommended routine use of ARMs followed by PEEP after induction of general anesthesia, and in the face of declining hemoglobin saturation [125]. Figure 38.23 Airway pressure and flow curves and dynamic pressure–volume (P–V) loops recorded during volume‐controlled ventilation (VCV) (9100c nxt, GE Healthcare) in a 23 kg dog (ideal body weight) anesthetized with isoflurane. A. The ventilator was set to deliver a VT (Vc) of 10 mL/kg (230 mL) at a respiratory frequency (FR) of 12 breaths/min and a 1:2 inspiration‐to‐expiration ratio (I:E). The end‐inspiratory hold was set to 40%. Peak inspiratory pressure (Ppeak), plateau pressure (Pplat), minute volume (VM = expired VT × respiratory frequency), and respiratory system compliance [Compl = expired VT/(Pplat – PEEP)] are shown on the left‐hand side of the screen. Compliance measurements are quasistatic because the duration of inspiratory pause was between 0.5–2 s (0.66 s). The inspiratory limb of the dynamic P–V loop shows a quasilinear increase in airway pressure during VCV (arrow). The P–V loop stored when the same animal was under pressure‐controlled ventilation (PCV) shows that the inspiratory limb has a more rounded shape during PCV (arrow). The slope of the P–V loop is shown by the dashed red line. B. Screenshot recorded after the cuffed endotracheal tube was kinked in the same animal under VCV. The airway pressure tracing is suggestive of increased Raw and there is an increased gradient between peak (15 cmH2O) and plateau pressure (9 cmH2O). The dynamic P–V loop shows increased expiratory resistance (area above the slope of the P–V loop – dashed red line). The slope is decreased because Ppeak increased with increased Raw. Quasistatic CRS remained constant (35–36 mL/cmH2O, or 1.5 mL/cmH2O/kg) after partial obstruction of the endotracheal tube. Despite the potential benefits of ARMs followed by PEEP, some individuals may not respond favorably to an open‐lung approach (Fig. 38.25) and the potential benefits should be weighed against the negative physiological impact of high alveolar pressures on hemodynamic function [126]. Elevated airway pressures (Palv) during an ARM increase intrathoracic (Ppl) and transpulmonary pressures (gradient between alveolar [Palv] and pleural pressure [Ppl]), reducing venous return through the vena cava [127,128]. In addition, distension of the alveolar wall by elevated Palv leads to compression of pulmonary capillaries and increases pulmonary vascular resistance (PVR)/RV afterload, which may further contribute to the decrease in stroke volume/cardiac output caused by the ARM [127–129]. For these reasons, this technique cannot be applied to hemodynamically unstable or hypovolemic individuals and close hemodynamic monitoring is necessary during lung recruitment maneuvers. Furthermore, ARMs may need to be interrupted and the target increase in airway pressure may not be achieved if cardiovascular compromise develops (e.g., severe hypotension). Inotropic/vasopressor drugs and/or intravenous fluid boluses may be initiated in anticipation of the hemodynamic compromise in animals that present arterial blood pressures that are approaching the lower acceptable range (60 and 90 mmHg for mean and systolic arterial pressure, respectively). Figure 38.24 Influence of body condition score (underweight, 1–4; normal, 5; overweight, 6–9) and thorax shape (intermediate shaped [Interm.] and barrel‐shaped [Barrel]) on CRS during volume‐controlled ventilation in anesthetized healthy dogs (n = 100). The VT (8–12 mL/kg) was set according to actual body weight and an inspiratory pause of 20% was added after the preset VT was achieved. Source: Reproduced from Asorey et al. [118], with permission. *Significant difference between groups (p < 0.05). Figure 38.25 Effects of an alveolar recruitment maneuver followed by open‐lung positive end‐expiratory pressure (ARM + PEEP) on the distribution of lung aeration considering two possible phenotypes: A. responder to lung recruitment; B. nonresponder to lung recruitment. Source: Adapted from Pensier et al. [126]. The degree of alveolar collapse leading to decreased CRS and oxygenation impairment varies substantially among mammalian species and there is no evidence supporting the routine use of ARMs in veterinary anesthesia. Incidence of hypoxemia is high in dorsally recumbent horses undergoing elective surgeries, with reports showing that up to 20% of dorsally recumbent animals receiving conventional mechanical ventilation develop severe intraoperative hypoxemia (PaO2 < 60%) while breathing 100% oxygen [2]. Oxygenation impairment observed in dorsally recumbent horses is caused by the development of a large shunt fraction, which may exceed 30–50% of pulmonary blood flow, due to atelectasis of dependent lung regions and these effects may persist despite the use of conventional mechanical ventilation [1,24]. Although recruitment maneuvers may be useful in this scenario, one must be aware of the cardiovascular depressant effects caused by the high airway pressures (60–80 cmH2O) that may be necessary to improve PaO2 in equine species [130], and of the possibility of such elevated pressures to cause alveolar overdistension/inflammation in horses [131]. In anesthetized horses subjected to an ARM reaching a Ppeak of 60 cmH2O, histological analysis of lung tissue revealed overdistended lung areas but there was no cytological (bronchoalveolar lavage) or histopathological evidence of lung injury [131]. However, compared to the groups of conscious horses and spontaneously breathing anesthetized horses, an increased expression of pro‐inflammatory cytokines (interleukin 1‐β and 6) matrix metalloproteinases (MMP 1 and 9) was observed in the lung tissue of groups that received conventional mechanical ventilation alone or combined with an ARM, suggesting that mechanical ventilation per se might be associated with early stages of lung injury [131]. Despite controversies regarding its clinical application, lung recruitment maneuvers should be considered to improve arterial oxygen levels in severely hypoxemic horses (PaO2 < 60 mmHg) undergoing surgery in dorsal recumbency [130,132–134]. If a decision has been made to apply an ARM in horses with colic, this procedure should be performed after abdominal decompression/exposure of the large colon, which may allow some improvement in CRS and PaO2 levels. In contrast to the situation in horses, the degree of oxygenation impairment caused by atelectasis is significantly less during routine anesthesia in small animal species. Currently, the use of ARMs followed by PEEP in small animal anesthesia may be considered in selected clinical conditions associated with poor CRS due to atelectasis and low PaO2/FIO2 ratio (< 300 mmHg) or hypoxemia. These conditions may be caused by obesity, increased intra‐abdominal pressure (e.g., laparoscopic surgery under pneumoperitoneum), diaphragmatic hernia, or presence of poorly aerated areas due to lung disease. Recruitment maneuvers can be performed in several ways as illustrated in Fig. 38.26. The simplest way of performing lung recruitment in humans involves a temporary increase in airway pressure to 30–40 cmH2O lasting up to 30–40 s, while monitoring the patient for adverse effects such as hemodynamic compromise [101,135] (Fig. 38.26A). A temporary increase in airway pressure to 40 cmH2O for 20 s, followed by 5 cmH2O PEEP has been successfully applied to increase arterial oxygenation and static CRS in healthy dogs undergoing laparoscopy [136]. Much higher airway pressures (60–80 cmH2O for 10–12 s), have been reported to recruit alveoli and promote an increase in PaO2 levels in dorsally recumbent horses undergoing elective or emergency (colic) surgeries [130,134]. Continuous positive airway pressure (CPAP) is a tool originally designed for non‐invasive ventilation in non‐intubated, spontaneously breathing patients. However, if the anesthesia ventilator has the option for performing CPAP, this ventilation mode may be selected in intubated patients to achieve an elevated airway pressure for a short period of time (e.g., 30 cmH2O for 20 s), before returning to a standard mechanical ventilation protocol and post‐recruitment PEEP, which aims to keep the alveoli opened [38,101,136] (Fig. 38.26B). However, most anesthesia machines do not have the option for CPAP. In this case, with the spontaneous mode selected, the rebreathing bag is manually squeezed with the pop‐off (APL) valve closed/semi‐closed to achieve the target airway pressure for the desired length of time. This technique may be used to improve oxygenation and expand the lungs in small animal species (dogs and cats) with diaphragmatic hernia. In this case, the increase in airway pressure should be applied after reduction of the visceral contents herniated into the thoracic cavity, while the surgeon is guiding the increase in airway pressure by visually assessing lung expansion within the thoracic cavity (see discussion below regarding re‐expansion pulmonary edema). The maximum airway pressure can be limited to 30 cmH2O or less, pending the severity of cardiovascular depression (e.g., hypotension) observed during the recruitment maneuver. Cats with a diaphragmatic hernia may be more sensitive to the negative cardiovascular effects of ARMs, therefore a reduced maximum airway pressure is initially recommended (≤ 20 cmH2O). Figure 38.26 Diagrammatic representation of airway pressure–time curves of alveolar recruitment maneuvers (ARMs). A. Manual recruitment with bag squeezing. B. Recruitment with continuous CPAP. C. Cycling maneuver with decremental positive PEEP trial. D. Stepwise VT changes. Effective alveolar recruitment should decrease the driving pressure (∆P, gradient between inspiratory and end‐expiratory pressure) and increase CRS. CPAP, continuous positive airway pressure; PEEP, positive end‐expired pressure; VT, tidal volume; CRS, respiratory system compliance. Source: Partially modified from Ball et al. [101]. Alveolar recruitment may also be performed using cycling maneuvers, which have three distinct phases: (1) hemodynamic conditioning; (2) recruitment; and (3) decremental PEEP trial (Fig. 38.26C and 38.27). During the hemodynamic conditioning phase, before the lung’s opening pressure is achieved, inspiratory pressures and PEEP are progressively increased with ventilation in PCV mode while the driving pressure (gradient between Pmax and PEEP) is held constant. Theoretically, pulmonary tissue stress is less than during CPAP maneuvers. Stepwise increases in airway pressure may reduce the possibility of overdistension of ventilated lung areas as it progressively spreads across the collapsed lung tissue, opening atelectatic alveoli in a more controlled manner than with CPAP maneuvers [101,135]. Stepwise increases in airway pressures also identify individuals with occult hypovolemia, who may not tolerate the higher airway pressures that are necessary to open collapsed lung areas [135]. If severe hypotension is identified during this phase, the procedure is interrupted, and intravenous fluid boluses and/or sympathomimetics are administered before the cycling maneuver is reinstituted. During the recruitment phase, while PEEP is maintained at the maximum target level (e.g., 15 cmH2O for 30 s), the driving pressure can be temporarily increased by adjusting Pmax to achieve inspiratory pressures that are necessary to open collapsed lung areas (e.g., 30 cmH2O). The decremental PEEP trial is the last phase of a cycling maneuver and aims to determine the lowest PEEP level that will prevent de‐recruitment or re‐collapse of lung tissue. As PEEP is progressively reduced in 2 cmH2O steps, it will reach the closing pressure, that is the pressure at which alveoli collapse [135]. Identification of the closing pressure can be performed by measuring the quasistatic CRS at the end of each stepwise decrease in PEEP during VCV with an inspiratory pause. The post‐recruitment PEEP level can be adjusted based on the PEEP that resulted in highest quasistatic CRS during the decremental PEEP trial + 2 cmH2O [135,137–139]. In general, greater cardiovascular depression occurs in anesthetized animals receiving positive‐pressure ventilation compared to anesthetized animals that are permitted to breathe spontaneously. Mechanical ventilation alters cardiovascular function directly through changes in intrapleural and alveolar pressures. Cardiovascular function is also indirectly modified by mechanical ventilation via changes in PaCO2 levels. A decrease in V̇E due to low respiratory frequency and/or low VT set on the ventilator will cause varying degrees of hypercapnia. In conscious dogs and humans, hypercapnia increases endogenous catecholamine levels leading to a cardiovascular stimulant effect (increased heart rate, cardiac output, and arterial blood pressure, and decreased systemic vascular resistance [SVR]). Although general anesthetics can potentially blunt the sympathetic stimulation caused by high PaCO2 levels, studies in anesthetized mechanically ventilated horses have shown that hypercapnia increases circulating plasma epinephrine and norepinephrine levels and results in cardiovascular stimulation [140,141]. In humans under general anesthesia, mild hypercapnia (PaCO2 up to 60 mmHg) during mechanical ventilation increases subcutaneous tissue oxygen tension, which may contribute to oxidative killing of surgical pathogens by neutrophils and reduce the rate of surgical infection [142]. However, cardiovascular depression/poor responsiveness to exogenous catecholamines can occur in the face of severe acidemia (pH < 7.2). In most patients, mechanical ventilation depresses cardiovascular function by decreasing venous return. The blood flow entering the heart through the vena cava (VR) is directly proportional to the gradient between the mean circulatory filling pressure (MCFP) of the venous circulation and right atrial pressure (RAP) divided by the resistance to blood flow in the venous circulation. The MCFP is determined by the stressed volume (Vs), which represents the blood volume that exerts pressure inside the veins and effectively contributes to VR; while the unstressed volume (Vus) is the blood that keeps small veins and venules minimally opened and does not contribute significantly to VR [143,144]. Changes in VR during spontaneous breathing and during mechanical ventilation are illustrated in Fig. 38.28. During spontaneous breathing, VR transiently increases because the gradient between MCFP and RAP increases as Ppl become more negative during inspiration. In contrast, mechanical ventilation decreases the gradient between MCFP and RAP as Ppl increases during inspiration. According to the Frank–Starling mechanism, as VR and preload are decreased, RV stroke volume decreases on the next heartbeat. Under normal circumstances, decreases in preload/stroke volume induced by mechanical breaths are relatively small. However, in hypovolemic animals, the inspiratory rise in Ppl caused by mechanical ventilation induces larger decreases in VR/preload, which may result in significant cardiovascular depression. An excessively large VT delivered by the ventilator may also induce greater decreases in VR/preload, mimicking the effects of hypovolemia. In addition to the effects on VR, distension of the alveolar wall caused by the rise in alveolar pressure (Palv) increases RV afterload because of compression of pulmonary capillaries in West zones I and II. The increase in RV afterload opposes RV ejection and may contribute to the decrease in RV stroke volume (Fig. 38.29) [116,145]. On the left side of the circulation, increases in pleural (Ppl) and alveolar (Palv) pressures induced by mechanical breaths cause intermittent increases and decreases in aortic blood flow and left ventricular (LV) stroke volume (Fig. 38.29). Aortic blood flow and LV stroke volume transiently decrease because of the inspiratory decrease in preload/RV stroke volume as discussed before; however, the maximum decrease in aortic flow/LV stroke volume caused by this mechanism will only be detected a few heartbeats later, during expiration, reflecting the time needed for blood to travel through the pulmonary circulation. On the other hand, an inspiratory increase in aortic flow/LV stroke volume occurs because of increased LV preload and decreased LV afterload caused by increased Palv and by increased Ppl, respectively, as illustrated in Fig. 38.29. Because patients that are hypovolemic have larger variations in aortic flow/LV stroke volume induced by mechanical breaths, heart–lung interactions during mechanical ventilation provide the physiologic basis for assessment of fluid responsiveness, that is, the determination of whether a patient will respond or not to intravascular volume expansion with increases in stroke volume/cardiac output [116,145]. Figure 38.27 Example of a cycling maneuver followed by decremental PEEP trial in anesthetized dogs. A. Progressive increases in Pmax and PEEP are performed in PCV mode (hemodynamic conditioning phase). Each step may last for a fixed number of breaths (e.g., 5 breaths). After a maximum PEEP level is achieved, the driving pressure (Pmax – PEEP) is increased to achieve the lung’s opening pressure (recruitment phase). After lung recruitment, progressive decreases in PEEP are performed with a fixed VT in VCV mode with an inspiratory pause lasting ≥ 0.5 s (decremental PEEP trial phase). Quasistatic CRS, measured after 1 minute at each stepwise decrease in PEEP, is used to identify the lowest PEEP associated with maximum CRS. After a new recruitment was performed, anesthesia was maintained in VCV + PEEP associated with maximum CRS + 2 cmH2O (open‐lung PEEP). B. and C. In healthy dogs anesthetized under VCV a cycling maneuver (ARM + open‐lung PEEP) resulted in higher quasistatic CRS and lower ∆P than animals anesthetized under VCV + PEEP (5 cmH2O) and VCV + ZEEP. PEEP, positive end‐expired pressure; Pmax, maximum airway pressure; PCV, pressure‐controlled ventilation; VT, tidal volume; VCV, volume‐controlled ventilation; CRS, respiratory system compliance; ARM, alveolar recruitment maneuver; ZEEP, zero end‐expired pressure; ∆P, driving pressure. Source: Data compiled from García‐Sanz et al. [138]. Precise monitoring of VT and airway pressure during positive‐pressure ventilation is critical because inappropriate mechanical ventilator settings can cause lung damage. Currently, it is recognized that there are four basic mechanisms that contribute, either alone or in combination, to lung injury associated with mechanical ventilation: barotrauma, volutrauma, atelectrauma, and biotrauma. Barotrauma, initially defined as excessively high airway pressures/Palv causing lung damage, was the first proposed mechanism of ventilator‐induced lung injury (VILI). However, experimental studies in the 1980s showed that VILI occurs when excessively high airway pressures/Palv causes excessive alveolar expansion (volutrauma) [146,147]. Therefore, elevated inspiratory pressure (equivalent to Palv) is more likely to result in VILI if it results in excessive increases in lung volume. This fundamental concept was shown by experimental studies demonstrating that mechanical ventilation with high airway pressures (Ppeak of 45 cmH2O) did not result in evidence of lung damage/inflammation when lung expansion was limited by low chest wall compliance (Fig. 38.30) [146]. Barotrauma is currently defined as lung injury caused by high transpulmonary pressures, that is the pressure difference between inside (Palv) and outside the lung (Ppl), which effectively results in alveolar overdistension [148]. Although barotrauma may occur during VCV because of high inspiratory pressures, the anesthesiologist should consider that elevated inspiratory pressures (high Palv) may not result in excessive increases in transpulmonary pressure (alveolar overdistension) if Ppl is also increased because chest wall compliance is markedly reduced, as in morbidly obese animals or animals with marked increases in intra‐abdominal pressure. As an example, standard mechanical ventilator settings (VT of 12–15 mL/kg) in horses with colic and severe abdominal distension may, on some occasions, result in Ppeak values that exceed 45 cmH2O. Although no specific measurements of transpulmonary pressures have been performed in horses under these circumstances, the decrease in Ppeak that is noticeable after the surgeon removes the distended bowel from the abdominal cavity suggests that the cause of vof the chest wall by abdominal viscera. Therefore, high inspiratory pressures under these conditions are less likely to be harmful to the lungs. Figure 38.28 Diagrammatic representation of inspiratory changes in venous return (VR) during spontaneous and mechanical ventilation. A. During spontaneous ventilation, the decrease in Ppl during inspiration increases right atrial transmural pressure (the difference between pressure inside and outside an elastic organ) and transiently decreases RAP. Venous return increases because the gradient between MCFP and RAP is increased. B. During mechanical ventilation, the increase in Ppl during inspiration decreases right atrial transmural pressure and RAP is increased. Venous return decreases because the gradient between MCFP and RAP is reduced. C. Greater decreases in VR are induced by mechanical ventilation in hypovolemic patients because the decrease in MCFP (due to a decrease in Vs) further reduces the gradient between MCFP and RAP. D. Mechanical ventilation with a large VT can mimic the effects of hypovolemia on VR and preload. A supraphysiological VT results in larger increases in Ppl, and causes greater increases in RAP, which further reduces VR. Vs, stressed volume; Vus, unstressed volume; VR, venous return; MCFP, mean circulatory filling pressure; RAP, right atrial pressure; Ppl, pleural pressure. Figure 38.29 Heart–lung interactions during mechanical ventilation. Mechanisms that cause intermittent decreases and increases in aortic blood flow/LV stroke volume are described in sequences A1 to A5 and B1 to B4, respectively. The increase in Ppl induced by mechanical breaths increases RAP (A1), which leads to a decrease in VR and RV preload (A2). PA blood flow and RV stroke volume are decreased (A3). The impairment in VR induced by mechanical ventilation is magnified by hypovolemia, which decreases the stressed blood volume (A4). The increase in Palv induced by mechanical breaths causes compression of capillaries not engorged with blood (West zones I and II) and increases RV afterload (A5), contributing to further decreases in PA blood flow/RV stroke volume (A3). The maximum decrease in aortic blood flow/LV stroke volume is detected during expiration because of the delay associated with transit of blood flow through the pulmonary circulation. Increased Palv squeezes blood out of capillaries in West zone III (B1) and increases venous return to the left heart (B2). Increased Ppl during inspiration (B3) decreases LV systolic transmural pressure (the pressure difference between the inside and the outside of LV during systole), consequently decreasing LV afterload (B4). The increase in LV preload (B1 and B2), combined with the decrease in LV afterload (B3 and B4) increases LV stroke volume during inspiration. Ppl, pleural pressure; RAP, right atrial pressure; VR, venous return; RV, right ventricle; PA, pulmonary artery; Palv, alveolar pressure; RA, right atrium; LA, left atrium; LV, left ventricle. Source: Adapted from Michard et al. [116]. Figure 38.30 Effects of mechanical ventilation with peak inspiratory pressures of 15, 30, and 45 cmH2O on capillary filtration coefficient (Kfc) in the lungs of rabbits with normal chest wall compliance (Closed chest, white bar) and in rabbits where lung expansion was restricted by a full body plaster cast surrounding the chest wall and abdomen. The body cast was molded while rabbits were breathing spontaneously (Body cast, black bars). The increase in Kfc was observed only when elevated airway pressures resulted in increased lung volume (Closed chest group), which likely resulted in high transpulmonary pressures (Palv – Ppl). Source: Reproduced from Hernandez et al. [146], with permission. * Significantly higher than 15 cmH2O in Closed chest group. † Significantly higher than Body cast group at the same peak inspiratory pressure. Besides barotrauma and volutrauma, atelectrauma, and biotrauma are now recognized as mechanisms of VILI [148]. Atelectrauma is caused by high shear forces from cyclic opening and closure of atelectatic alveoli, which can trigger a local inflammatory response and injure the alveolar–capillary membrane [148]. The alveolar instability caused by the intermittent opening and closure of atelectatic lung areas, named “tidal recruitment,” occurs when the level of inspiratory pressure is large enough to open atelectatic alveoli, but these alveoli subsequently collapse during expiration because the level of end‐expiratory pressure is insufficient to keep them open throughout the ventilatory cycle [149–151]. Biotrauma has been recognized as an important mechanism of VILI in ARDS. It is characterized as a biological response, including activation of a pro‐inflammatory cytokine cascade that promotes injury even in lung areas not subjected to mechanical stress and in extrapulmonary organs [148]. Although data are lacking in veterinary medicine, it has been recognized that a lung‐protective ventilation strategy in humans with ARDS, aimed at minimizing mechanical stress to the lungs by use of low VTs, may attenuate the systemic inflammatory response and associated multiple organ failure, contributing to a decrease in mortality [123,152–154]. Experimental animal models of ARDS show that application of PEEP with a relatively small VT is less injurious to the lung than ventilation with a large VT in the absence of PEEP [147]. In the medical field, the importance of VILI and its negative impact on clinical outcomes was first established in humans with ARDS [123,153–155]. Historically, routine anesthesia in humans was performed with relatively high VT (10–15 mL/kg) without PEEP in most instances [156]. While the concept of lung‐protective ventilation with low VT (≤ 6 mL/kg) and high PEEP (> 12 cmH2O) has been a standard procedure for long‐term ventilation in humans with ARDS in the ICU setting for more than 20 years [157], ventilation strategies for routine anesthesia in humans only started to change with advances in the understanding of mechanisms of VILI, where lung damage is closely related to the amplitude of cyclic stretch of lung parenchyma induced by mechanical ventilation [158]. In human patients without lung disease undergoing anesthesia for elective surgeries, mechanical ventilation with lower VT (6 mL/kg) and 10 cmH2O PEEP may reduce lung inflammation compared to a higher VT (12 mL/kg) [159]. A reduction in VT from values ≥ 10 mL/kg to < 8 mL/kg also improves the clinical outcome (lower incidence of postoperative pneumonia/respiratory failure and shorter duration of hospital stay) in human patients without ARDS in the operating room [160,161]. To prevent postoperative pulmonary complications, current guidelines for protective mechanical ventilation in human patients undergoing surgery recommend targeting a VT of 6–8 mL/kg based on predicted (ideal) body weight and an initial PEEP level of 5 cmH2O [162]. Ventilator settings in veterinary species cannot be directly extrapolated from recommendations in humans for several reasons including: (1) minute ventilation (VT × f) should be adjusted according to the CO2 production (metabolic rate), which can vary among animals and man; (2) the VT adjusted on the ventilator may vary among species because of differences in airway deadspace A critical point to consider is the size of mechanical breaths delivered to veterinary patients because the VT must be large enough to overcome the airway deadspace (VDaw) and expand the alveoli (VTalv), according to the formula: Typically, the As explained in Chapter 37, CO2 removal by the lungs and PaCO2 levels depend on the fraction of VT that goes beyond the physiological deadspace Therefore, the rate of CO2 removal is better described by the effective alveolar ventilation per minute (V̇A) than by the minute ventilation (V̇E), because the former considers the Fig. 38.31 shows deadspace calculations using volumetric capnography in a dog anesthetized with isoflurane receiving mechanical ventilation (VCV mode). As discussed in Chapter 37, the Enghoff modification of the Bohr equation for calculating the physiological deadspace to tidal volume ratio (
38
Oxygen Therapy, Mechanical Ventilation, and Anesthetic Management of Patients with Respiratory Disease
Introduction
Oxygen therapy
Physiologic effects of oxygen therapy
) oxygen content difference is assumed to be 5 mL per 100 mL of blood, reflecting a normal cardiac output. The shunt bands have been drawn to include the range of hemoglobin (Hb) and PaCO2 levels shown. Note that at the higher levels of shunt flow (30–50%), there is little improvement in arterial oxygen levels even when the inspired oxygen concentration is 100%. Also note that if the inspired oxygen concentration and PaO2 are known, it is possible to obtain a quick estimate of the degree of venous admixture or shunt flow.
Strategies for oxygen supplementation
Low‐flow oxygen systems
High‐flow oxygen systems
Oxygen toxicity
Mechanical ventilation during anesthesia
Evolution of mechanical ventilators used in veterinary and human anesthesia
The bellows‐type ventilator
Influence of gas compression, breathing circuit compliance, and fresh gas flow on ventilator performance
Volume delivered by the bellows
FGF entering the breathing circuit
FGF/volume added during inspiration a
Breathing circuit compliance losses b
Gas compression lossesc
Inspired VT d
% difference between bellows and inspired VT
20 kg dog
300 mL
(15 mL/kg)
1 L/min
(16.6 mL/s)
33.3 mL
9 mL
(Ppeak = 10 cmH2O)
30 mL
(Ppeak = 10 cmH2O)
294 mL
(14.7 mL/kg)
−2%
2 L/min
(33.3 mL/s)
66.6 mL
10.8 mL
(Ppeak = 12 cmH2O)
36 mL
(Ppeak = 12 cmH2O)
320 mL
(16 mL/kg)
+7%
4 L/min
(66.6 mL/s)
133.2 mL
12.6 mL
(Ppeak = 14 cmH2O:
42 mL
(Ppeak = 14 cmH2O:
379 mL
(19.0 mL/kg)
+26%
3.3 kg cat
50 mL
(15 mL/kg)
1 L/min
(16.6 mL/s)
33.3 mL
8.1 mL
(Ppeak = 9 cmH2O)
27 mL
(Ppeak = 9 cmH2O)
48 mL
(14.5 mL/kg)
−4%
2 L/min
(33.3 mL/s)
66.6 mL
10.8 mL
(Ppeak = 12 cmH2O)
36 mL
(Ppeak = 12 cmH2O)
70 mL
(21.2 mL/kg)
+40%
4 L/min
(66.6 mL/s)
133.2 mL
16.2 mL
(Ppeak = 18 cmH2O)
54 mL
(Ppeak = 18 cmH2O
113 mL
(34.2 mL/kg)
+126%
Modern anesthesia ventilators
Modes of mechanical ventilation utilized during anesthesia
Continuous mandatory ventilation
Volume‐controlled ventilation (VCV)
Pressure‐controlled ventilation (PCV)
Dual‐controlled ventilation
Assisted and assisted‐controlled ventilation modes
Pressure support ventilation
Volume‐controlled ventilation
Pressure‐controlled ventilation
Dual‐controlled ventilation
Breathing pattern
Only mandatory breaths
Only mandatory breaths
Only mandatory breaths
Termination of inspiratory flow
Time cycled
Time cycled
Time cycled
Inspiratory flow pattern
Constant (square wave) flow
Decelerating flow
Decelerating flow
Target variable
VT
Pmaxa
VT
Advantages
Allows maintenance of V̇E and PaCO2 regardless of changes in CRS and Raw
If an inspiratory pause is added, quasistatic CRS and Raw (Ppeak − Pplat) can be assessed
Lower airway pressures +/− better compliance than VCV
Combines the advantages of VCV and PCV
Disadvantages
Higher airway pressures (Ppeak) than PCV
V̇E and PaCO2 influenced by changes in CRS and Raw
Cannot assess Raw by the gradient between Ppeak and Pplat (typical Ppeak in VCV not measured)
Cannot assess Raw by the gradient between Ppeak and Pplat (typical Ppeak in VCV not measured)
Spontaneous intermittent mandatory ventilation
Monitoring pulmonary mechanics during mechanical ventilation
Pressure–volume (P–V) loops
Compliance of the respiratory system and airway resistance
Strategies for reducing atelectasis during mechanical ventilation
Effects of mechanical ventilation on cardiovascular function
Ventilator‐induced lung injury
Mechanical ventilation during anesthesia in humans and veterinary species
and physiological space
, which lead to differences in effective alveolar ventilation (V̇A); and (3) the maximum airway pressure and driving pressure (gradient between Pplat and PEEP) may differ among animals and man because of differences in CRS, which is affected by body mass (large versus small animals), shape of chest wall, intra‐abdominal volume/pressure, and degree of atelectasis, among other factors. Tidal volume adjustments however should be based on ideal (predicted) body weight as variable degrees of alveolar overdistension/higher driving pressures may occur if VT calculation is based on actual body weight in obese animals [163]. In animals with poor body condition scores, calculation of VT based on actual body weight instead of ideal body weight could result in alveolar sub‐distension/atelectasis.
measured by the single nitrogen washout (Fowler’s) technique in adult humans is 150 mL (2 mL/kg for an adult averaging 75 kg) [164]. Therefore, a VT between 6–8 mL/kg (450–600 mL for 75 kg adult human) is large enough to overcome the deadspace represented by the conducting airways, effectively expanding the alveoli during inspiration without causing alveolar overdistension or cyclic alveolar opening and closure (tidal recruitment), especially when combined with a moderate PEEP level (5 cmH2O or more). However, a VT currently recommended for routine ventilation during anesthesia in humans (e.g., 6 mL/kg) may not be large enough to go past the VDaw measured in dogs by volumetric capnography (6.5 to 7.6 mL/kg) and effectively expand the alveoli [165].
, which is the sum of areas of the respiratory system that do not participate in gas exchange, including the conducting airways
and alveoli that are ventilated but not perfused (alveolar deadspace
):
:
/VT) may overestimate true
in the presence of increased areas of low alveolar ventilation‐to‐perfusion (V̇A/Q̇) ratio and shunt. The PaO2/FIO2 ratio close to 500 mmHg (480 mmHg) in Fig. 38.31 demonstrates that venous admixture/shunt is minimal; therefore, the Bohr–Enghoff formula may provide a reasonable estimate of true
/VT. For this dog,
was 7 mL/kg (6.4 mL/kg of
and 0.6 mL/kg of
) and mechanical ventilation with a standard VT
Stay updated, free articles. Join our Telegram channel
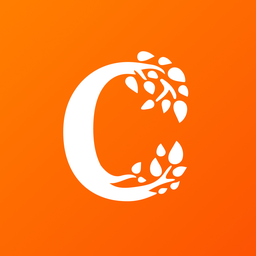
Full access? Get Clinical Tree
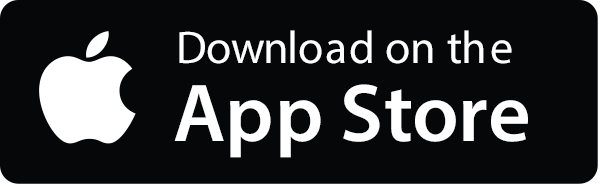
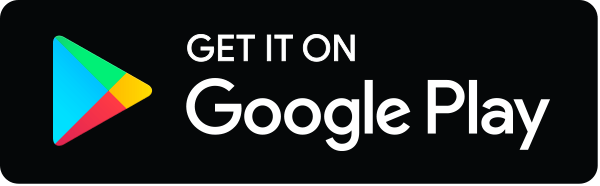