Fig. 2.1
Confocal image of cryptochrome (CRY) immunoreactivity in the Procambarus clarkii brain at 19:00. The image corresponds to optical sections of a whole-mount preparation. Neurons of the protocebral anterior medial cluster (AMC) express strong CRY immunoreactivity. Bars 100 μm. (Modified from Fanjul-Moles et al. 2004)
Cryptochromes were originally identified in plants and have orthologues and paralogues among insects and vertebrates (Yu et al. 2010). The role of cryptochromes in the circadian clock differs among species. In mammals, CRY1 and CRY2 represent the core of the circadian oscillator with a light-independent function (Lin and Todo 2005); however, in Drosophila and other insects, CRYs were originally thought to act as circadian photoreceptors. Recently, in non-drosophilid insects, a second cry (cry2) gene has been identified in some species that encodes for a light-insensitive protein; this is more similar in sequence to the mammalian CRYs that act as transcriptional repressors (Zhu et al. 2005 ). Other insects, such as bees and beetles, possess only a mammalian-like CRY (Yuan et al. 2007).
Drosophila CRY also appears to have a tissue-specific pleiotropic role, because in clock neurons that generate rhythmic locomotor behavior it acts as a photoreceptor and mediates light synchronization of the circadian clock by promoting the light-dependent degradation of dTIM (Emery et al. 1998; Peschel et al. 2009). After absorbing a photon, dCRY undergoes a conformational change involving its C-terminal domain and binds to dTIM, which is then tagged for ubiquitination and proteasomal degradation. The mechanism by which dCRY initiates the cascade of events that leads to dTIM degradation remains unclear (Dubruille et al. 2009). However, the homology between Drosophila CRY and photolyases, or light-dependent enzymes utilizing a flavin cofactor to repair DNA, suggests that flavin-dependent reduction/oxidation redox reactions may be involved in dCRY functions (Froy et al. 2002). Cryptochrome-bound flavin is found in an oxidized redox state in vivo, and light activation results in flavin photoreduction to a radical intermediate that represents the likely signaling state. The derived photocycle of animal cryptochromes is therefore similar to the reaction mechanism of plant cryptochromes (Fig. 2.2). Both photocycles involve the reduction of flavin, leading to a cycling between the reduced (active) and oxidized (inactive) redox forms (Berndt et al. 2007; Hoang et al. 2008).
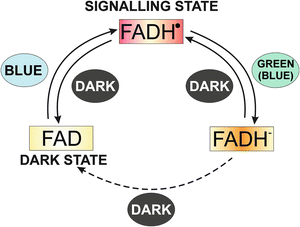
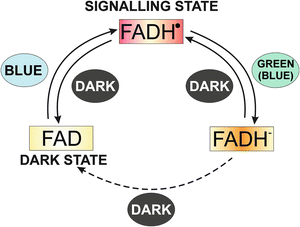
Fig. 2.2
The photocycle of plant cryptochromes. In the dark, the flavin chromophore is in its oxidized state. Blue light induces conversion to a metastable semi-quinone redox state that is the activated signaling state. Green light causes further reduction to the fully reduced redox state of flavin, which is inactive in signaling. In the dark, fully reduced flavin reoxidizes to the fully oxidized form and can be reactivated by blue light. The photocycle of plant cryptochromes is different from DNA photolyases, in which only the fully reduced redox state is catalytically active. (Reproduced with permission from Hoang et al. 2008)
In Drosophila it has been proposed that CRY activation involves intramolecular electron transfer and presumably subsequent conformational changes; thus, the cellular redox status also regulates the transfer of photic information and CRY stability (Lin et al. 2001). Using a microarray analysis, Sathyanarayanan et al. (2008) identified three thioredoxin domain-containing redox molecules (GstE7, Txl, and CG11790) and a cytochrome p450, CYP49a1, that modulate light-dependent CRY and TIM degradation. These results suggest that cellular redox status and electron transfer modulate the light-dependent activation of CRY, which in turn affects the subsequent transmission of the light signal to TIM and the degradation of CRY itself, with the subsequent clock resetting.
In zebrafish cells, the light-induced redox changes stimulating intracellular mitogen-activated protein kinase (MAPK) signaling that transduces photic signals to zCry1a gene transactivation (Hirayama et al. 2009). Importantly, light also drives the production of intracellular ROS, such as H2O2, that leads to an altered redox status and increases intracellular catalase activity by stimulating catalase transcription, an event which occurs after the maximum expression of the zCry1a gene has been reached (Uchida et al. 2010). This increased catalase activity diminishes light-induced cellular ROS levels, resulting in decreased zCry1a transcription and creating a negative feedback loop. Thus, this altered redox state triggers the transduction of photic signals that regulate and synchronize the circadian clock (Uchida et al. 2010) (Fig. 2.3).
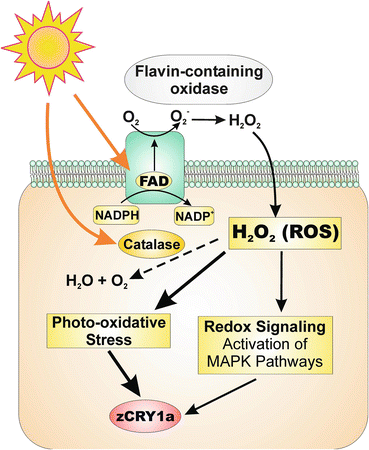
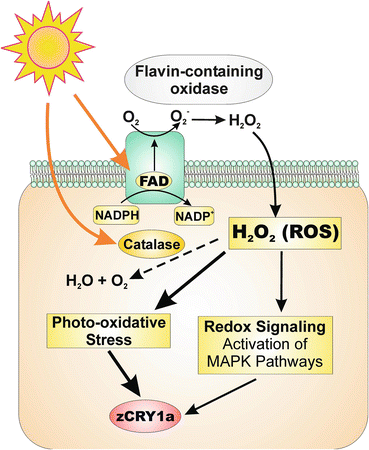
Fig. 2.3
Potential molecular mechanism underlying light-dependent redox signaling in zebrafish. In the presence of flavin-containing oxidases, light drives the production of intracellular reactive oxygen species (ROS) such as H2O2. Excess ROS production has deleterious effects because ROS can react with various cellular targets to cause photo-oxidative stress. However, light-induced ROS can also take on a signaling role by stimulating MAPK pathways that lead to transcriptional activation, including transactivation of the zCry1a gene. Light also increases catalase transcription and thus intracellular catalase activity, resulting in H2O2 degradation and decreased photo-oxidative stress. This reduction in ROS also leads to decreased zCry1a expression, thus creating a negative feedback loop that directly impinges on the circadian clock. (Reproduced with permission from Hirayama et al. 2009)
2.3 Cryptochromes, Circadian Rhythms, and Redox Mechanisms in Crustaceans
In crustaceans, some behavioral studies have reported rhythmic activity–rest changes as well as synchronization or entrainment of circadian rhythms by blue light (Fanjul-Moles et al. 1992; Bernal-Moreno et al. 1996; Miranda-Anaya and Fanjul-Moles 1997; Aguzzi et al. 2009, 2011). Recently, it has been reported that positive phototaxic circadian rhythmicity is stronger under blue light in the parasite Argulus japonicus (Branchiura) (Yoshizawa and Nogami 2008), suggesting the presence of cryptochromes in the circadian photoreceptor system of different groups of crustaceans. The circadian system of genus Procambarus is considered to be distributed throughout a multioscillatory system in a hierarchical nature (Fanjul-Moles and Prieto-Sagredo 2003 ). It is a complex model, which includes three pairs of coupled oscillators such as the retina, the eyestalk X-organ–sinus gland complex (XOSG), putative brain pacemakers, and probably a pair of extra-retinal photoreceptors in the caudal abdominal ganglion. A pair of extra-retinal photoreceptors in the brain (BPR) are involved in light-dependent entrainment (Strauss and Dircksen 2010) (Fig. 2.4), which in crayfish, similar to insects, appears to be mediated by CRYs (Miranda-Anaya and Fanjul-Moles 1997) (Fig. 2.5).
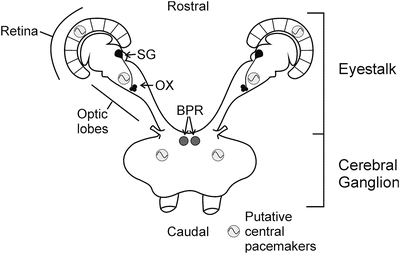
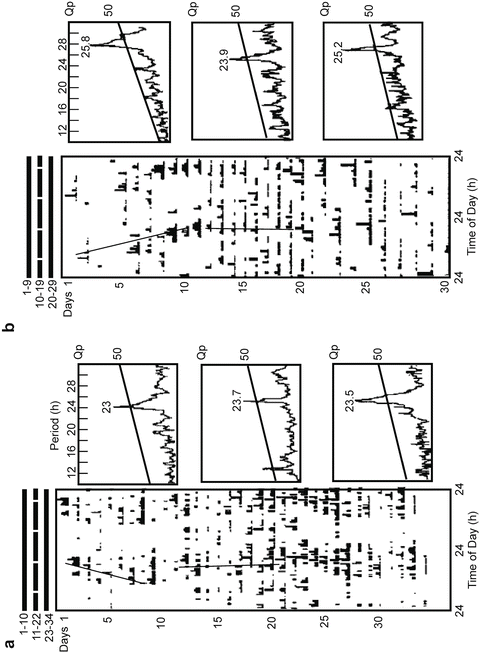
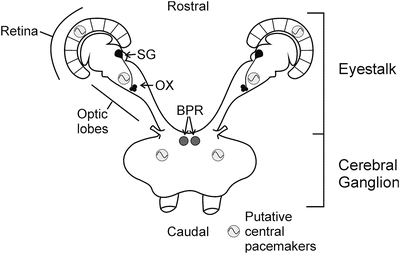
Fig. 2.4
Schematic summary of known circadian oscillators and their functional correlations in crayfish. Established physiological oscillators or pacemakers (grey circles) exist in the retina, the X-organ–sinus gland complex, and likely the brain, whereas the brain photoreceptor (BPR) is not an oscillator but is responsible for light-driven entrainment of locomotory rhythms. BPR brain photoreceptor, SG sinus gland, XO X organ
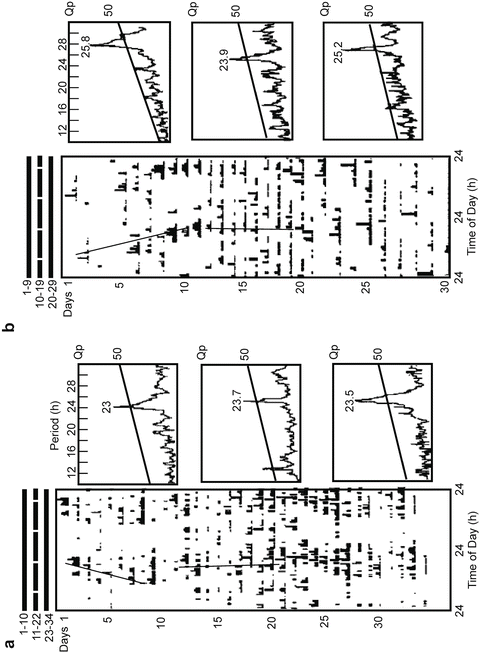
Fig. 2.5
Locomotor activity record and its associate periodograms of two juvenile crayfish, intact (a) and retina ablated (b), that were exposed to red Skeleton photoperiod (SP) (two 30-min pulses of 640 nm and 25 W m2). Note the “lights-on” peak during the entrainment condition in the intact crayfish is not present in retina-deprived crayfish. Note the rhythm splitting as well as the change of the rhythm period value during the DD post entrainment. (a) A 19:00 pulse light occurring at CT 14 during the 11th day evokes a phase delay. (b) A 19:00 light pulse occurring at CT 13 produces an advance. Regression line periods are 22.8 and 24.1 h (a) and 25.3 and 23.88 h (b), respectively. (Modified from Miranda-Anaya and Fanjul-Moles 1997)
Immunochemical, biochemical, and behavioral studies using a Drosophila anti-CRY antibody (Fanjul-Moles et al. 2004; Escamilla-Chimal and Fanjul-Moles 2008; Sullivan et al. 2009) have shown the presence and circadian rhythm of CRY in the brain of crayfish, as well as its role in this organism in the activity of rhythm synchronization. The molecular characteristic of this protein has not been characterized. Based on both the homologies and the molecular mass detected by Western blot analysis (60 kDa), and considering that the peptide sequence within the C-terminus of Drosophila sp. used to generate the antibody was specific to the Drosophila melanogaster and Drosophila pseudoobscura CRY 1, this protein seems to function as a photopigment in the brain of crayfish (Escamilla-Chimal and Fanjul-moles 2008; Sullivan et al. 2009). Recently, Mazzotta et al. (2010) isolated and cloned a new CRY gene from the Antarctic krill (Euphausia superba). The EsCRY gene appears to be an orthologue of mammalian-like CRYs and clusters with the insect CRY2 subfamily. Importantly, these results suggest the presence of two CRYs in crustaceans, similar to mammals and some insects. Both CRYs could act either as a photopigment or as a transcription factor participating in the transcription–translation loop (TTL) of the core of the clock.
Both the mechanisms of the photo-activation of CRY and the different pathways involved in the resetting of the clock are unknown in crustaceans; however, the results of some experiments provide hints toward ROS and redox mechanisms underlying these processes. Fanjul-Moles et al. (2009) demonstrated bi- and unimodal daily and circadian rhythms in all glutathione (GSH) parameters, substrates, and enzymes in the putative pacemakers of crayfish, including the optic lobe, brain, and retina, as well as an apparent direct effect of light on these rhythm conditions, especially in the retina.
In a series of experiments we used two different species, Procambarus clarkii and Procambarus digueti, adapted with a different geographical distribution and different latitudes, 27° and 17° N, with different luminosity and daylength throughout the year. During the summer P. clarkii is exposed to photoperiods of as long as 16 h whereas in southern latitudes the maximal daylight for P. digueti is about 13 h. Using animals of both species collected in the field, we performed different ecophysiological studies in our laboratory (Prieto-Sagredo et al. 2000; Fanjul-Moles and Prieto-Sagredo 2003).The results of different experiments showed daily and circadian changes in OS produced by different light conditions are counterbalanced by antioxidant circadian rhythms of glutathione and related enzymes detected in liver and hemolymph. The robust antioxidant circadian rhythms of P. clarkii are able to entrain to all conditions, resetting to lights on or off. However, the weak circadian glutathione system of P. digueti did not entrain to LD cycles, showing a random distribution of phases (Fig. 2.6). This observation suggests that the photoperiodic history of each species determines the adaptive abilities of entrainment to extreme light conditions, probably by means of the OS control of circadian antioxidative mechanisms. This finding, of course, indicates interesting ecological implications in the process of light synchronization. Moreover, we found circadian differences between liver and hemolymph glutathione (GSH) oscillatory systems that indicate differences in the coupling strength of both compartments to the crayfish circadian system. These results led us to propose the hemolymph as a passive system responding to an oscillatory driving force depending on various self-sustaining oscillators, one of which could be the liver, proposed elsewhere as a peripheral clock in mammals (Stokkan et al. 2001).
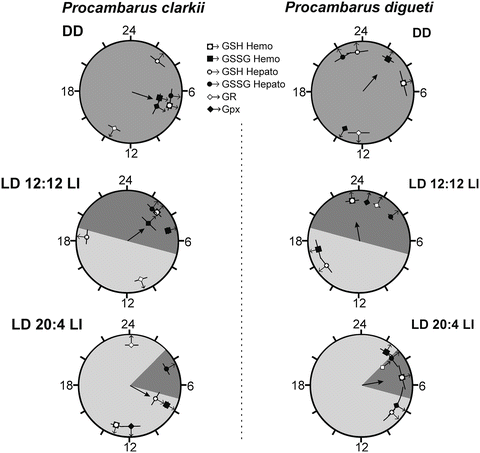
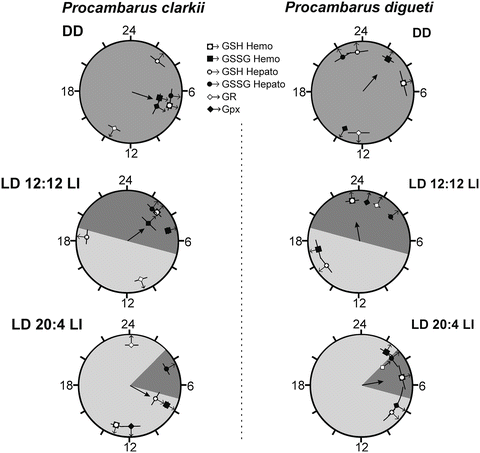
Fig. 2.6
Estimated acrophases of the glutathione daily rhythms of Procambarus clarkii and P. digueti entrained to the control and experimental conditions. The mean vector indicates the clustering direction of the rhythms acrophase. Horizontal bars indicate the mean ± 6 SE. V test indicated, for P. clarkia, P < 0.05 in LD 12:12 LI and LD 20:4 LI. (Modified from Fanjul-Moles and Prieto-Sagredo 2003)
Herein we investigated the retina and optic lobe–brain (OL-B) circadian GSH system and its ability to handle reactive oxygen species (ROS) produced as a consequence of metabolic rhythms and light variations. We characterized daily and antioxidant circadian variations of the different parameters of the glutathione system, including GSH, oxidized glutathione (GSSG), glutathione reductase (GR), and glutathione peroxidase (GPx), as well as metabolic and lipoperoxidative circadian oscillations in the retina and optic lobe–brain complex (OL-B) proposed elsewhere as central pacemakers of crayfish, determining internal and external GSH system synchrony. The results of our experiments demonstrated bi- and unimodal daily and circadian rhythms in all GSH cycle parameters, substrates, and enzymes in OL-B and retina, as well as an apparent direct effect of light on these rhythms, especially in the retina. The luminous condition appears to stimulate the GSH system to antagonize ROS and lipid peroxidation (LPO) daily and circadian rhythms occurring in both structures, oscillating with higher LPO under dark conditions.
These results confirmed that light producing photo-oxidation is a factor that determines ROS in crayfish, as was previously reported (Duran-Lizarraga et al. 2001). Putative pacemakers in the retina and eyestalk–brain complex showed higher GSH/GSSG ratio mean values in LD than in DD. The increment in this ratio in the retina and eyestalk coincides with the photophase, indicating that OS produced by light is antagonized by the rapid transformation of GSSG into GSH. This result suggests that the antioxidant defense system (ADS) is upregulated at the mid-photophase level, between ZT4 and ZT6 at the same time of the CRY higher decrement (Fanjul-Moles et al. 2004, 2009; Escamilla-Chimal and Fanjul-Moles 2008) (Fig. 2.7). This response suggests a photo-oxidative redox stress signal similar to those of the model of light-induced signaling cascades potentially involved in the control of the circadian clock of zebrafish (Uchida et al. 2010). In crayfish, the redox signal might be the result of the direct effect of ROS produced by the light onset of the LD cycle on CRY to reset the clock. This ROS increment seems activate the glutathione system, especially glutathione reductase (GR), directly or indirectly, through an unknown signaling pathway (Fanjul-Moles et al. 2009). It has been proposed that light-induced ROS, especially in response to UVB, is involved in the activation of mitogen-activated protein kinase (MAPK) downstream antioxidant-response effectors (Assefa et al. 2005), and certain MAPK signal regulation by GSH has been described recently in the mouse (Limón-Pacheco et al. 2007). Many reports have indicated that the GSH/GSSG ratio is an important “sensor” for ADS regulation (Dalton et al. 1999; Dalle-Donne et al. 2009; Maciel et al. 2010), occurring in parallel with ROS increment. These changes promote the oxidation of protein cysteinyl thiols, which activate or deactivate specific enzymes in the signaling cascades (for a review, see Maciel et al. 2010).
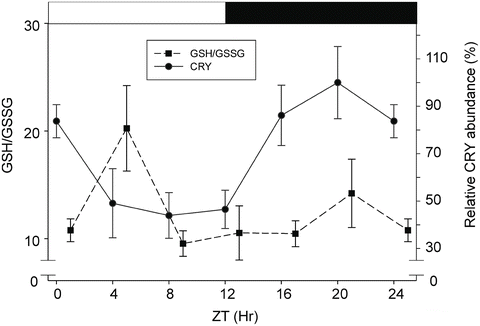
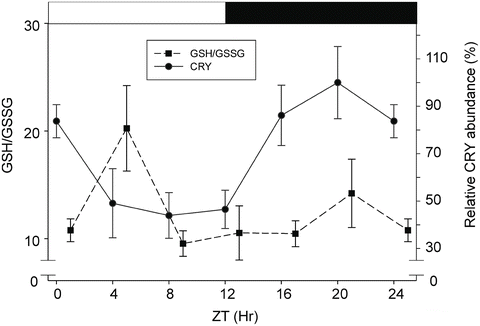
Fig. 2.7
Relationship between GSH/GSSG ratio and CRY abundance in the brain of P. clarkii submitted to a 24-h LD cycle. Light drives photo-oxidative stress, as is shown by the low GSH/GSSG ratio, and the decrease in CRY abundance suggests CRY degradation. However, importantly light seems to upregulate glutathione reductase (not shown), which increases GSH and the GSH/GSSG ratio. This reduction in reactive oxygen species (ROS) leads to greater CRY degradation. The abundance increments of CRY occur in the dark phase (from ZT 12 to ZT 20) when the brain showed maximal oxidation (not shown). ZT zeitgeber time. (Modified from Escamilla-Chimal and Fanjul-Moles 2008 and Fanjul-Moles et al. 2009)
As already mentioned, the photic input to the clock directly activates MAPK signaling cascades in zebrafish cells. The light-induced activation of these pathways controls the expression of two evolutionarily related genes, z64Phr and zCry1a, revealing that light-dependent DNA repair and entrainment of the circadian clock share common regulatory pathways (Hirayama et al. 2009). We are exploring similar possibilities in crayfish, where we have already found the presence and cycling of MAPK proteins, such as extracellular-signal-regulated kinases (ERK), in some of the putative pacemakers of this animal, such as brain and eyestalk (unpublished data), as well as the presence of PKA and its possible participation in the circadian regulation of cAMP response element-mediated circadian gene expression (unpublished data).
2.4 Synchronization and Metabolism
Another stimulus able to reset the phase of the circadian system depends on the modulation of the redox state through metabolism (Bianchi 2008). Experimental evidence supports the link between metabolism and circadian rhythms in mammals. The timing of metabolism can be influenced by the circadian system via systemic cues from the suprachiasmatic nuclei (SCN) or peripheral oscillators. A relationship between both processes by means of the AMP/ATP ratio, sensed mainly by adenosine monophosphate-dependent protein kinase (AMPK) or glucose and fatty acid sensors, has been shown (Albrecht 2012). Furthermore, it is well documented that the circadian clock controls the level of many cellular and circulating metabolites, and it is accepted that there is a cyclic relationship between the circadian clock and metabolism wherein the rhythm impacts metabolism and metabolism feeds back to impinge upon the rhythm (Roennnenberg and Merrow 1999). Interestingly, AMPK impacts the mammalian circadian clock mechanisms in various ways. It can directly phosphorylate CRY1, leading to destabilization and degradation of this core clock protein, and consequently affecting the negative limb of the circadian clock mechanism, or it may modulate PER2 protein stability via an indirect mechanism involving casein kinase ε (CK1ε). (Lamia et al. 2009) Thus, AMPK appears to be another potential regulator of the coupling and interaction between metabolism and the circadian clock, as it is in the case of light.
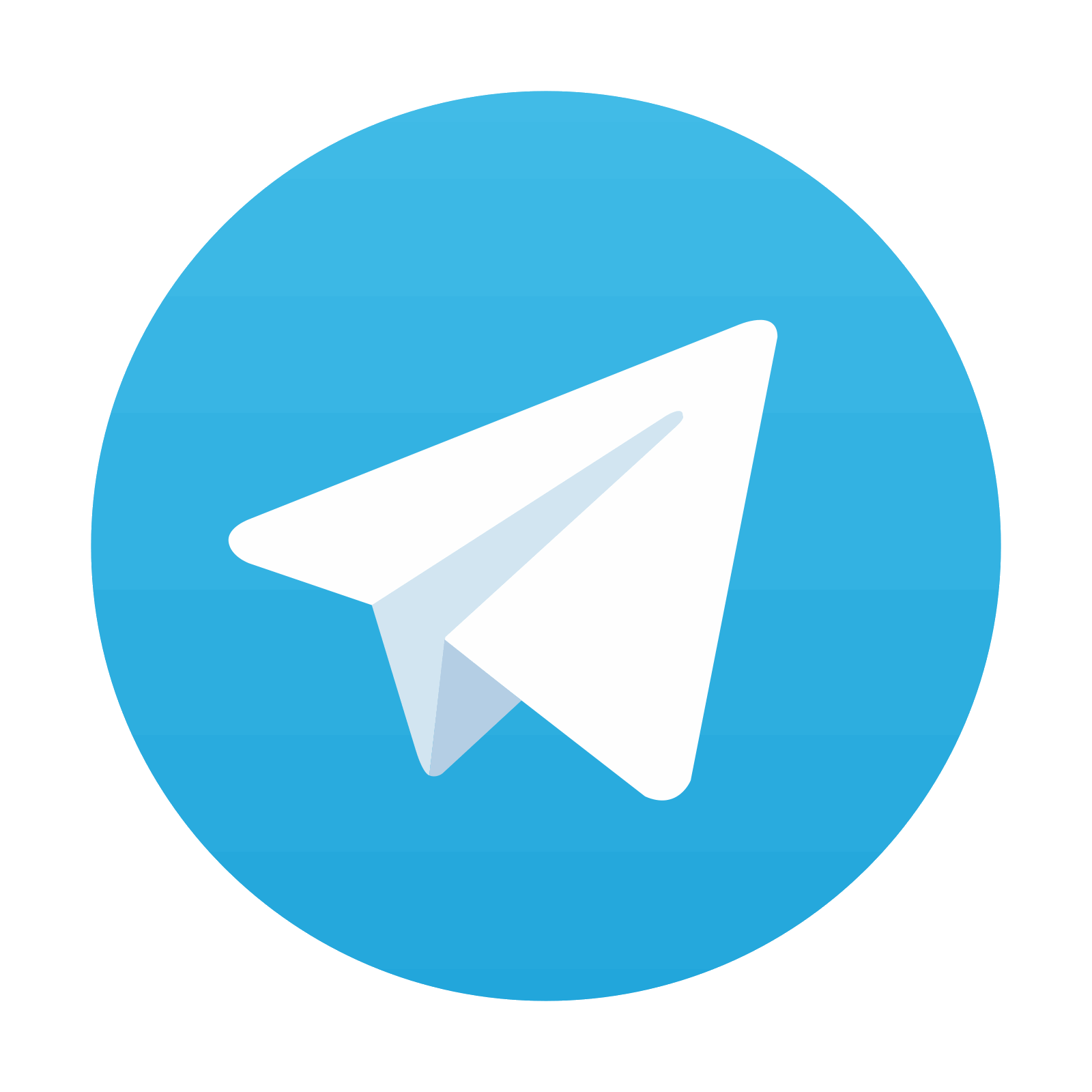
Stay updated, free articles. Join our Telegram channel
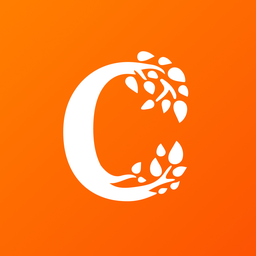
Full access? Get Clinical Tree
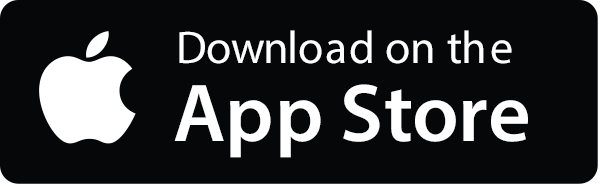
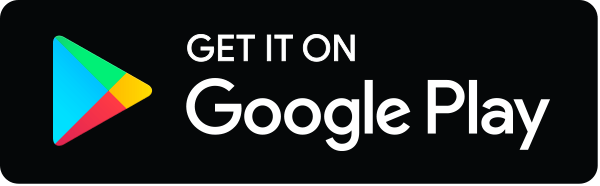