Rebecca J. Webb VetSurg, Ventura, CA, USA Fractures are common in veterinary medicine and often require surgical fixation for the return of limb function. The fixation of fractures requires implants, which can either be internal (Steinmann pins and Kirschner wires [K‐wire], bone plates, and interlocking nails [ILNs]) or external (external skeletal fixators [ESFs]). Internal fixation has the benefit of providing stable fixation to the fracture due to the proximity of the implants to the bone. Depending on the construct, either primary or secondary bone healing will occur. Internal fixation, however, requires a surgical approach to place, which can damage the surrounding tissues and lead to delays in healing. In comparison, ESFs have the advantage of being able to be placed without a surgical approach to the fracture, which has a distinct advantage for preserving the soft tissues and vasculature of the fracture site. They can be modified easily postsurgery and are relatively simple to remove when they are no longer needed. Due to their external nature, they are not as stiff and strong as other internal fixation options, such as plates, and complications, such as pin loosening, can occur over time. External fixators require more intensive postsurgical care in the form of bandaging, which may not be tolerated by some patients and clients. In this chapter, we will discuss the appropriate use and application of each type of implant along with tips and tricks for the smooth application of each. Steinmann pins and Kirschner wires (K‐wires) are long pins made of 316L stainless steel. These pins and wires come in a variety of sizes, and, typically, Steinmann pins refer to pins larger than 0.062 in. and K‐wires refer to pins 0.062 in. and smaller (Figure 39.1). These implants are very versatile and have a wide range of uses for orthopedic fixation. These pins can be used as intramedullary implants to resist bending at the fracture site, to secure small fragments by skewering them, in a cross‐pin fashion, to maintain temporary reduction of the fracture while a stronger primary fixation is applied, or as components of an external skeletal fixation. Pins are mostly used to resist bending forces, and the strength of a pin is calculated by the radius of the pin to the fourth power. Due to this, small increases in the size of a pin will have a significant increase in the strength of the pin when resisting bending. Figure 39.1 Kirschner wires and Steinmann pins of various sizes within an autoclavable cloth bag. Source: © Rebecca Webb. Pins can be placed within the intramedullary canal to provide resistance to bending forces. Placement of these pins can either be performed in a retrograde (advancing the pin from within the fracture site to the outside of the bone, then redirecting across the fracture site) or a normograde (advancing the pin into the intramedullary canal from the proximal aspect of the bone and across the fracture site) manner. The bones that are amenable to intramedullary pinning include the humerus, femur, tibia, ulna, and metacarpal and metatarsal bones. Intramedullary pinning of the radius is contraindicated due to the risk of carpal joint injury. The size of the pin used will be determined by the medullary canal size of the patient’s bone. Clinicians should aim for at least 70% fill of the medullary canal when the IM pin is the only device within the intramedullary canal. Pins can also be used in conjunction with a plate to increase the overall strength of the construct (see “Plates ‐ Bridging Plates”). In these instances, the size of the pin needs to be reduced to allow bi‐cortical screw purchase, so clinicians should aim to fill 35–40% of the medullary canal in these cases (Figure 39.2).1 The size of the medullary canal can either be judged at the time of surgery or using calibrated radiographs to make measurements of the canal for pre‐surgery fixation planning. Figure 39.2 Postoperative craniocaudal (a) and lateral (b) radiographs of internal fixation of a left chronic comminuted tibia fracture with a 2.8 mm IM Steinmann pin and a 3.0 mm Arthrex® OrthoLine™ plate. Source: © Rebecca Webb. IM pins will resist bending forces but have limited strength against rotational or compressive forces. For this reason, IM pins are rarely used alone as a fixation, and they are commonly combined with additional implants, such as cerclage wire, plates, or as part of an external fixator, to counteract these other fracture forces. The use of multiple IM pins, referred to as “stacked pinning,” was historically used to improve the resistance to rotational forces; however, it was found that multiple IM pins provided no improvement in resistance when compared with a single pin, so the application of stacked pinning is no longer recommended. 2 Prior to the wide availability of plating systems, intramedullary pinning, in addition to placement of cerclage wire, was historically used frequently for fracture repair. While this can be an efficacious treatment option, careful case selection is imperative to its success. This type of fracture fixation is suitable only for reconstructable long oblique or spiral fractures. In these fracture types, there must be complete reconstruction of the bony column; this provides load‐sharing between the IM pin and cerclage and the bone and gives stability to the overall construct. The placement of an intramedullary pin can be in either a normograde or retrograde manner. Pins placed within the tibia are typically normograde to avoid accidental injury to the stifle joint surface. Placement within the other bones mentioned above can be performed in either a normograde or retrograde fashion. Normograde is typically preferred when possible, as there is less disruption of the fracture callus for insertion of the pin. However, normograde insertion can be challenging to accurately perform if visualization of the entry point cannot be visualized, such as in the femur. It is important prior to placement of the pin that the surgeon has two pins of the same length so that one of the pins can be used as a comparison measuring pin to monitor how deep the pin in the bone is being inserted. The pin can be advanced into the fracture fragments with either a hand‐driven Jacobs chuck or with power equipment. Using a hand chuck typically will give the surgeon a better feel for the insertion of the pin and help the surgeon judge if they are still within the medullary canal by the ease with which the pin is advanced. Power equipment can also be used, but surgeons are more likely to over‐advance the pin or penetrate through the cortex when it is used, as there is little feedback through the power equipment for the surgeon. Once the pin is visualized at the fracture site, the pointed tip of the pin can be cut off to help prevent accidental penetration through the cortex into the distal joint. The surgeon should be aware that if the tip is removed from the IM pin being placed, the reduction in length is taken into consideration when using the comparison measuring pin (or the measuring pin reduced by a similar amount in length to make them the same again) to judge penetration into the distal fragment. The fracture is then reduced, and the pin advanced into the distal fragment. Once the pin starts to engage within the distal metaphyseal bone, the pin will become subtly more difficult to advance again. This is a good indicator to the clinician that the pin length is sufficient. Pin length should be checked against the measuring pin one more time, and the distal joint should be put through a range of motion to feel for crepitus. The presence of crepitus or restricted range of motion of the joint indicates joint penetration and should be rectified prior to leaving the OR. If this is noted, the pin should be withdrawn from the distal fragment and advanced again in a new position within the intramedullary canal. Simply pulling the pin back and away from the joint without redirecting it is not advised, since with micromotion at the fracture site, the pin will likely just advance back through the hole and into the joint again postsurgery. Once the additional fixation (plate, external fixator, or cerclage) has been applied to stabilize the construct, the tip of the pin should be cut as short as possible. This is most easily performed by dissecting away some of the soft tissue from the exposed pin length to advance the pin cutters to cut the pin as close to the bone as possible. If it is not possible to apply the pin cutters close to the bone, the surgeon can withdraw the pin 1–2 cm from its insertion within the bone. To be accurate during the withdrawal, it is recommended to score the pin with pin cutters so the clinician can tell how much of the pin has been withdrawn from the bone. Once the pin is slightly withdrawn, the surgeon can cut the pin shorter than before. The pin should be cut as close as possible to the insertion, then the pin is advanced back into its original location within the distal metaphysis with a pin punch (Figure 39.3). This method allows for a shorter length of pin exposed, which is important, as leaving the end of the pin too long will lead to complications, such as seroma, muscular trauma, pin loosening/migration, poor use of the limb, and nerve damage (e.g., in femoral fractures with sciatic nerve impingement). Small K‐wires are used for the fixation of small bony fragments. This is commonly performed when repairing avulsion fractures, such as tibial tuberosity avulsions or olecranon fractures (Figure 39.4). When placing these pins, the pins should be placed with a bridge of bone separating them, and when used alone as the fixation, the pins should be slightly angled away from each other to improve fixation strength. More than one pin, typically two, should be placed, or the small fragment will just rotate on the singular pin. When placing pins to repair growth plate fractures, the ideal angle is typically perpendicular to the growth plate, so that any further remaining growth from the growth plate can occur (Figure 39.5). Figure 39.3 (a,b) Pin punch. The tip of the punch is roughened to allow it to capture the head of the pin. A mallet is used on the other end to advance the pin within the bone. (c) Craniocaudal radiograph of a postoperative femur fracture repair with an IM pin and lateral 3.5 mm Synthes LCP® plate. Note the IM pin is well‐seated within the femur with no pin protruding past the greater trochanter. Source: © Rebecca Webb. Figure 39.4 (a) Pre‐ and (b) postoperative radiographs of an immature dog with a left tibial tuberosity avulsion fracture. Small 0.045″ K‐wires have been placed with a 20‐gauge figure‐8 tension band to secure the tuberosity bone fragment. Source: © Rebecca Webb. Figure 39.5 Postoperative craniocaudal radiograph of surgical repair of a capital physeal fracture in a medium‐sized dog. Note the pins are placed perpendicular to the physis to help limit any impact on further physeal growth. Source: Courtesy of Dr. Desmond Tan. Figure 39.6 IMEX® Veterinary K‐wire benders. These can be useful for bending small K‐wires at acute angles. If these are not available, the surgeon can use a metal Frazier tip suction piece, Jacob’s chuck, or needle drivers. Source: © Rebecca Webb. Figure 39.7 Source: © Rebecca Webb. For fractures in the distal metaphysis with only a small amount of bone stock for implant purchase, cross‐pinning is an appropriate technique. Depending on the size of the patient (and, therefore, the size of the bone), either K‐wires or Steinmann pins can be used. The overall strength of this fixation is low, and due to that, this technique is best suited to patients who are expected to have rapid healing (young, otherwise healthy patients). To place these pins, the fracture is reduced, and a pin is placed from the distal segment in a lateral‐to‐medial direction and a second pin in a medial‐to‐lateral direction. It is important for both pins to penetrate the far cortex to give stability to the construct. The pins should cross within the metaphyseal segment and not within the fracture (Figure 39.8). Occasionally, during the placement of cross pins, the pin will fail to penetrate the far cortex and, instead, start to travel up the shaft of the bone. This has been termed a “Rush‐pin” technique and has been found to have less stability than traditional cross‐pinning.3 This typically occurs when the angle of placement is acute and a small pin is used, allowing it to bend instead of penetrating the far cortex. This fixation is commonly used to repair physeal fractures. Given that the pins are not placed perpendicular to the growth plate, there is some concern that they will impede further growth from the physis. However, it is important to note that the physis has already been significantly damaged during the incident causing the fracture, so it is challenging to determine if the physeal disturbance is secondary to the trauma or the repair. When these fractures occur in superficial locations, pin removal once the fracture is healed can be considered if there is evidence of physeal dysfunction. Figure 39.8 (a) Pre‐ and (b) postoperative radiographs of a left distal radial Salter‐Harris type II fracture and distal ulna greenstick fracture in a Yorkshire terrier. The postoperative image shows two 0.035″ K‐wires placed in cross‐pin fashion. Note that the pins cross within the metaphyseal bone and not within the fracture site. Source: © Rebecca Webb. Orthopedic wire is often used in conjunction with pins or a plate in reconstructable fractures. It is made from 316L stainless steel, which has been fashioned into a malleable wire that comes in a range of sizes from 16‐ to 24‐gauge (Figure 39.9). The wire comes in two different forms, either gauge wire, which is straight wire that comes on a spool, or loop wire, which has an eye created in one end. Orthopedic wire can be used in a variety of techniques, including cerclage, tension band, hemicerclage, and interfragmentary. As cerclage and tension bands are the most common uses, we will focus on their application in this chapter. Orthopedic wire resists loads aligned with the wire well but has very little bending strength; due to this, it needs to be applied correctly for the construct to gain strength from the wire. As with IM pins, the strength of orthopedic wire is calculated by its radius to the fourth power, which leads to a dramatic increase in the strength of the wire for a small increase in wire size. It is recommended to use the largest size that appears appropriate for the size of the bone of the patient. In the author’s practice, a general guideline for size selection is 16‐gauge wire for patients over 40 kg, 18‐gauge wire for patients over 20 kg, 20‐gauge wire for patients weighing 10–20 kg, and 22‐ and 24‐gauge for small dogs and cats. However, ultimately, the size chosen will depend on the bone size and the forces applied to it. Figure 39.9 Orthopedic wire of different sizes on spools: 18G, 20G, and 22G. Source: © Rebecca Webb. Orthopedic wire can be secured in a variety of ways, and in veterinary patients, twist knot, single‐loop knot, and double‐loop knot are most commonly used. The twist knot is the most common, since the two types of loop knots require a tensioner for placement. When the three‐knot types were compared biomechanically, the double loop cerclage had a higher tension capacity than twist and single loop knots and was able to resist a greater load.4 Clinically, any of these knots can be used with success so long as the principles of placement are adhered to. Figure 39.10 Twist knot using 18‐gauge orthopedic wire. The twist knot on the left exhibits the correct application of a twist knot: note how the two strands are both twisted around each other. The twist knot on the right shows the incorrect application of a twist knot with one strand wrapped around the other. This gives the appearance of a “snake on a pole” and is not an appropriate twist. If this occurs, the knot should be restarted with a new piece of wire, and the surgeon should focus on applying traction to the wire as the wire is twisted. Source: © Rebecca Webb. Figure 39.11 Jaws of the wire twister centered in the middle of the twist. To complete the twist, the surgeon pulls firmly away from the bone while continuing to twist the wire ends. Source: © Rebecca Webb. Cerclage is a technique that involves wrapping orthopedic wire around the bone tightly to apply compression to the fragments and give stability (Figure 39.12). This technique is only useful when the fracture is reconstructable and should only be used in long oblique or spiral fractures or to protect a fissure from propagating. Cerclage is only functional when the fracture fragments contact each other, and the bone column is reconstructed. If the fragments are poorly reduced or the fragments shift at all following placement, the wire will become loose and fail to function. When well‐placed, cerclage should not impair fracture healing.6 However, when cerclage is placed poorly or the fragments shift following placement, it becomes loose. It is thought that the loose wire impedes the formation of blood supply to the fracture and subsequently impairs healing. Figure 39.12 Postoperative lateral radiograph of a right long oblique tibial fracture that has been stabilized with an IM pin and a 3.5 mm Arthrex® OrthoLine™ locking plate. 18‐gauge cerclage wire was used to prevent the propagation of fissures noted pre‐operatively proximally and distally. Source: © Rebecca Webb. Guidelines for appropriate cerclage wire placement are as follows: During the placement of cerclage wire around the bone, it is important that there is no interposing soft tissue. If there is soft tissue underneath the wire, the pressure of the wire after tensioning will cause necrosis of the underlying soft tissue, leading to loosening of the wire. To avoid this, a cerclage wire passer can be used to create a circumferential tunnel around the bone while excluding the surrounding soft tissue. Through the wire passer, the wire is then placed, enabling placement without entrapping soft tissue. In the absence of this instrument, a curved hemostat can be used in a similar manner. Avulsion fractures occur in regions where muscles originate or insert onto bone. The avulsed bone fragment is typically reduced with two or more K‐wires. The pull of the muscle that attaches to the bone fragments creates tension on the repair, which must be counteracted. In these cases, a tension band wire is placed to counteract the tension force and convert it into a compressive force across the fracture line. To place a tension band, the fragment is reduced with two or more K‐wires. These K‐wires should be placed perpendicular to the fracture line to be more effective and should be placed parallel to each other. A hole is drilled transversely across the main bone fragment to create a bone tunnel to pass the orthopedic wire. The placement of this tunnel is approximately equidistant below the fracture line, as the pins are above the fracture line. The orthopedic wire is threaded through this tunnel, and the ends cross over each other. One end is then looped around the pins to create a figure‐of‐8 pattern. The two ends are then tightened, typically with a twist knot, and the pins are bent and cut short as previously described (Figures 39.4 and 39.13). As the orthopedic wire makes a few sharp turns, it is important for the clinician to eliminate the slack as the wire is passed through each turn. This helps to ensure that the wire is tight on the bone and will be effective in its function. In larger gauge wire, this becomes more challenging to do. In these cases, the use of two twist knots, one on either side, can be helpful to eliminate the slack (Figure 39.14). Figure 39.13 16‐gauge orthopedic wire used for figure‐of‐8 tension band placement on a greater trochanter model with one twist knot. Two smooth 0.062″ K‐wires are placed to secure the fragment followed by the tension band. Source: © Rebecca Webb. Figure 39.14 16‐gauge orthopedic wire used for figure‐of‐8 tension band placement on a greater trochanter model with two twist knots. Two smooth 0.062″ K‐wires are placed to secure the fragment followed by the tension band. To create the second twist knot, a loop is made in the wire between the pins in the first section of the figure‐of‐8 pattern. The ends of the wire are twisted as normal to create two twist knots. Source: © Rebecca Webb. Plates and screws are commonly used in orthopedic surgery to provide stability to fractures. Locking and nonlocking implants are available with locking plates being preferred by many surgeons, particularly in comminuted fractures due to increased stability with their use. Recent advances in plate design include scalloping of the underside of the plate to decrease cortical bone contact and preserve periosteal blood flow, development of compression holes, combination locking and compression holes, variable angle locking screws, and the use of new materials, such as titanium alloys. Plates and screws can be used alone or in combination with other implants to stabilize complex fractures. The appropriate application of these implants depends on the fracture configuration and the method in which the plate is being used. In this chapter, we will discuss the basics of these implants and their uses, however, it is important to note that there are subtle nuances to each system and their application. Clinicians should be aware that there are many continuing education courses on these implants and their appropriate application, which can be of great benefit when starting to use a system that is unfamiliar to the clinician. Screws are versatile implants and can be used in multiple ways. Screws can be categorized in one of two ways. First, they are characterized by the type of screw (e.g., cortical, cancellous, locking, cannulated, and self‐tapping). Second, screws can be categorized as to how the clinician uses them in the patient (e.g., plate screw, positional screw, and lag screw). Screws are commonly made from 316L stainless steel. Titanium alloy screws are now available, but they should be reserved for use with titanium plates. Cortical screws are threaded along their length and are designed for implantation into dense cortical bone. The pitch of a screw describes the distance between the threads and is a common descriptor for the different types of screws. The pitch of a cortical screw is typically shorter than that of a cancellous screw but longer than that of a locking screw, and the depth of the threads is typically shallower than that of a cancellous screw but deeper than that of a locking screw (Figure 39.15). When cortical screws are implanted within a plate, they function by compressing the plate to the bone, and this creates friction between the bone and the plate to give stability to the construct. Due to this, it is important that when these screws are placed within a plate, they are tightened appropriately, or their function will be impaired. When using cortical screws, the clinician should be cautious that the plate is sitting flush against the bone prior to placement of the screw, as a large gap between the plate and bone will result in the screw pulling the bone toward the plate, disrupting the prior reduction of the fragments. Figure 39.15 From left to right: 4.0 mm cancellous screw, 3.5 mm cortical screw, and 3.5 mm locking screw. Note that the pitch of the threads is the smallest for the locking screw, followed by the cortical, then the cancellous screw. The depth of the threads of the cancellous screw is deepest, followed by the cortical, then the locking screw. Source: Courtesy of Christine A. Valdez. In comparison to cortical screws, cancellous screws have a thinner core diameter (compared with cortical screws of the same size) but wider and deeper threads (Figure 39.15). This increases the holding power of these screws within the trabecular bone of the metaphysis. These screws come in either completely threaded or partially threaded variations. The fully threaded variant functions like a cortical screw when placed in a plate. The partially threaded version can have a “lag effect” when placed in the traditional manner (see “Screw uses: Lag screw”), so long as the threads only engage the far fragment. Locking screws are screws that physically engage or “lock” into a plate. The locking mechanism to achieve this varies for different plating systems. Commonly, the screw head is threaded, and these threads engage into either matching threads within the plate (Locking compression plate [LCP®] by Synthes, OrthoLine™ by Arthrex®) (Figure 39.15) or to cut their own threads into the plate (PAX® Polyaxial locking system by Securos Surgical®). Additional locking systems include the FIXIN® (Intrauma®) and String of Pearls (SOP™) (Orthomed) systems. The FIXIN® system uses a threaded bushing within the screw hole and a conical taper to the screw head to lock the screw into the plate. The String of Pearls system uses cortical screws to lock into the plate hole or “pearl” using a thread at the base of the screw hole as well as a contact ridge within the screw hole. Locking screws give strength to a plate construct in a different manner than cortical screws. As the head of the screw is “locked” into the plate, they form a fixed angle construct, meaning that their position in relation to the plate and bone is fixed. These constructs do not rely on close contact between the bone and plate to create friction and give strength as cortical screws do. Instead, the forces created during the loading of the bone in locking constructs are converted into compressive forces at the screw‐bone interface to give strength (see “Locking plate systems”).7 Cannulated screws are screws with a central hole within the screw (Figure 39.16). Through this hole, a K‐wire can be threaded through. These screws are placed by first passing a K‐wire into the proposed location for the screw. This K‐wire acts as a guide for the final placement of the screw. Over this K‐wire, a cannulated drill bit is used to drill into the bone for the screw hole. The cannulated screw can then be placed over the K‐wire and into position. These screws are most helpful for reconstruction of metaphyseal or epiphyseal fractures. Achieving the appropriate angulation of screws for fixation of these fractures can be challenging. Cannulated screw systems give the clinician the ability to have multiple attempts to achieve the correct screw placement with the K‐wire prior to drilling the screw hole, which makes these screw systems invaluable for the repair of fractures where there is limited bone stock for implantation (Figure 39.17). Compression screws are placed across a fracture line and function to compress the near and far cortices together. They typically achieve compression across the fracture line due to a change in the pitch diameter of the threads of the screw with a wider pitch in the leading threads of the screw and a narrower pitch near the head of the screw. This means that when the screw is placed, it travels more quickly through the bone with the leading threads of the screw and then more slowly with the threads near the head. The change in the thread pitch results in compression between the two cortices during placement, similar to a screw placed in “lag fashion” (see “Screw uses: Lag screw”) (Figure 39.18). Figure 39.16 (a) A cannulated 3.0 mm headless compression screw with a K‐wire going through the center of the screw. Typically, the K‐wire is placed first, a cannulated drill bit is threaded over the K‐wire to drill the hole, and the screw is then placed over the K‐wire. (b,c) Pre‐operative (b) lateral and (c) craniocaudal radiographs of a juvenile canine patient with a lateral humeral condylar fracture. (d,e) Postoperative (d) lateral and (e) craniocaudal radiographs of the same patient postfixation with a 4.0 mm cannulated, partially threaded screw (Synthes) and an epicondylar 0.062″ K‐wire. (a) Source: Courtesy of Christine A. Valdez; (b–e) Source: Courtesy of Dr. Desmond Tan. The general steps for screw placement are as follows: Figure 39.17 Fixation of a Salter‐Harris type II fracture of the proximal tibia using a cannulated screw and K‐wires. (a) Pre‐operative craniocaudal radiograph of a young canine with a Salter‐Harris II fracture. (b) Intraoperative fluoroscopy was utilized in this case to perform the repair in a minimally invasive manner. In this image, a K‐wire is being placed from medial to lateral. Two additional small (0.045″) K‐wires have been placed in this case from distal to proximal to give additional fixation and secure the segment while the medial‐lateral screw is placed. (c) Once the surgeon was satisfied with the trajectory of the medial‐lateral K‐wire, the K‐wire was over‐drilled with a cannulated drill bit, and a 3.5 mm cannulated screw was placed. (d) Postoperative craniocaudal radiograph showing the final fixation with the medial‐lateral cannulated screw and the two distal‐proximal K‐wires. Source: © Rebecca Webb. The term “lag screw” relates to a method of screw placement and not a particular type of screw. In fact, any screw can function as a lag screw, as long as its threads only engage the far cortex. Since the threads of the screw only engage the far cortex, when the head of the screw contacts the near cortex and is then tightened, this creates compression across the two fragments in this region. This effect can be achieved using screws that are partially threaded or placing a fully threaded screw in a screw hole where the near cortex has been over‐drilled so that the threads do not engage the bone. This is called a “glide hole”. These screws need to be placed at a right angle to the fracture line to create compression across the fracture. This limits their effective use to fractures where the length of the fracture is greater than 1.5x the diameter of the bone. The following steps outline the placement of a fully threaded screw as a lag screw. Figure 39.18 A 3.0 mm headless compression screw. Note the wider pitch in the leading threads of the screw and a narrower pitch near the head of the screw, which function to apply compression across a fracture line or osteotomy. Source: Courtesy of Christine A. Valdez. A positional screw is used to secure a fragment back into position for reconstruction of a fracture. Positional screws are typically used when the placement of a lag screw would lead to the fragment collapsing into the medullary canal. To place these screws, the bone fragment is held in apposition with bone reduction forceps, and the screw hole is drilled in a traditional manner. Once the screw is placed, apposition of the fragments should be maintained. Unlike a lag screw, the threads of these screws engage both the near and far fragments. However, this is overall a less stable construct than a lag screw. These screws are typically used to reconstruct complex articular fractures. A plate screw is a screw placed to secure a plate to the bone. The size of the screw used to affix the plate will depend on the size of the plate (see “Plates”). Bone plates are most commonly made from 316L stainless steel, with some newer plates now made with titanium alloys. Bone plates are available in many different styles, each with their advantages and disadvantages. One of the biggest divisions in plating systems is between the nonlocking plates and the locking plates. Nonlocking plates, when placed, bring the plate in close contact with the bone. The contact between the bone and plate creates friction, and this friction creates stability to allow healing. Due to this, it is important that the plate is well‐contoured to the bone surface and that there is no soft tissue between the plate and bone. Locking plates function differently than nonlocking plates, and they instead create a fixed angle construct, which functions like an external fixator placed close to the bone. These plates do not rely on direct contact between the plate and the bone to create stability for healing. Locking plates are thought to create a stronger and stiffer construct compared with traditional plates.9 This may reduce the number of cortices needed for a stable construct. The angle‐stable nature of the screws also allows any monocortical screws placed to provide more benefit to the stability of the construct than nonlocking monocortical screws.9 Although locking plates are less reliant on accurate contouring for strength when compared to nonlocking plates, excessive gaps between a locking plate and the underlying bone will weaken the construct and are to be avoided. Bone plates are typically labeled by the size screw that the holes accept. The most common sizes include 1.5, 2.0, 2.4, 2.7, 3.5, and 4.5 mm, although other sizes are available in different plating systems. The most common shape for a bone plate is the straight plate. The manufacturers typically produce a range of different length plates in each size to minimize the need to cut the plates intraoperatively. Aside from the straight plates, a range of other shaped plates are produced, including the T‐plate, curved plates (for acetabular repair), and procedure‐specific plates, such as the pancarpal or pantarsal arthrodesis plates, distal femoral osteotomy plates, anatomic‐specific plates, such as the humeral condylar plate, tibial osteotomy plates, and pelvic osteotomy plates, among others. Depending on the fracture configuration and how a plate is applied to the bone, plates can function in several fashions. In long oblique, reconstructable fractures, a lag screw may be used to provide interfragmentary compression. However, this lag screw is not strong enough on its own to withstand all the forces applied to the fracture site. Neutralization plating is where a bone plate is applied in addition to a lag screw(s) to protect the lag screw(s) from failure, and it remains “neutral” by not applying compression to the fracture site. The neutralization plate protects the screw(s) from all rotational, bending, and shear forces. In nonreconstructable fractures, the plate is applied as a bridging plate. As the fracture has not been reconstructed, there is no load‐sharing between the plate and the bone, which means that the forces that enter the distal segment of the bone are transmitted from those distal screws and through the locking plate to the screws within the proximal segment of bone. The forces basically bypass the fracture zone, and the plate alone carries the full force in this location. Due to this non‐load‐sharing construct, the plate must withstand all forces until a mature callus forms. The plate‐rod combination can be particularly effective for these fractures, as the intramedullary pin is effective in protecting against bending forces and the plate is effective in protecting against axial compression and rotation. Typically, when an IM pin is used in combination with a plate, it is recommended that it fills 35–40% of the medullary canal.1 This size reduces strain on the plate and increases its fatigue life while still allowing microstrain within the fracture, which is beneficial for callus formation.1 In addition, the IM pin can also be helpful for the clinician to both re‐establish spatial alignment of the limb in comminuted fractures, as well as maintain reduction while the bone plate is placed. In these cases, the IM pin is placed first, followed by the plate. As the IM pin is occupying a portion of the medullary canal, some screws may need to be monocortical (only engaging one cortex). Due to this, the plate‐rod technique lends itself more to locking plates than nonlocking plate constructs. Additional methods for increasing the plate strength when used in a bridging fashion include selecting a larger plate, shortening the working length of the plate (by placing screws closer to the fracture site) or by adding an additional implant (such as an IM pin as previously discussed or a second plate).10 During weight‐bearing in metaphyseal shear fractures, there are compressive forces which occur. These can lead to a collapse of the articular surface. Buttress plates are placed to prevent this collapse. This method of plate use is uncommon in veterinary patients, as these fractures tend to be infrequent in our patients. Figure 39.19 An Arthrex® OrthoLine™ 3.5 mm broad plate. The second hole from the left is a compression hole. Note the sloped nature of the hole. When the clinician wishes to apply compression to the fracture site, the hole for the screw is drilled at the higher end of the hole (to the left of the image). Then, as the screw is tightened, the bone underlying the plate slides along the plate to apply compression. Source: © Rebecca Webb. Specific compression plates can be used to apply a compressive force across a fracture gap. This function should only be applied to transverse fractures; application of a compressive force across an oblique fracture will lead to a shear force across the fracture fragments, and this could lead to fragment displacement and loss of reduction. Compression plates have specifically designed holes that are oval rather than the traditional round holes. In addition, the hole is sloped, the slope is higher away from the fracture site and lower toward the fracture (Figure 39.19). The clinician drills an eccentrically placed hole (away from the fracture, at the high side of the sloped hole), and as the screw is tightened, the head slides down the slope to the lower end of the hole. During this, the bone fragment the screw is engaging also moves with it, thus creating compression at the fracture site. Compression can be applied from both sides of the fracture. The amount of compression applied is plate system and plate size specific. For example, in the Synthes dynamic compression plate (DCP) system, 1.0 mm of compression is applied for each compression hole in the 3.5 and 4.5 mm systems and 0.8 mm in the 2.7 mm plate system. After the insertion of one compression screw, additional compression can be applied by applying a second compression screw. This is performed by applying the second compression screw before the first is fully tightened. Compression is mostly used when reconstructing transverse fractures and during plating osteotomy procedures. As previously discussed (see “Cortical screws”), nonlocking plates are affixed to the bone with cortical screws. These screws compress the plate down to the bone. The friction created by the contact between the plate and the bone provides stability to the healing fracture. Nonlocking plates were some of the first bone plates produced, and they are typically less stiff and strong than their locking counterparts.9 Due to this, anatomic reconstruction of the fracture is usually recommended when using them, so that load‐sharing can occur between the bone and the plate construct, giving more stability to the fracture site and decreasing the risk of early implant failure. As these plates rely on bone‐to‐plate contact for stability, they need to be accurately contoured to the bone surface, so that when the cortical screws are applied and tightened, the bone fragments do not shift out of reduction. Nonlocking plates can have additional functions, such as compression holes, which are used to apply compression to the fracture or osteotomy site. The DCP is a nonlocking plate with the ability to apply compression to the fracture site. The plate was first introduced in 1969.11 The oval shape of the holes allows 25° of angulation of the screw in the longitudinal plane and 7° in the transverse plane. This plating system comes with three different drill guides. The green (neutral) drill guide will apply the drill hole centrally within the screw hole and produce a neutral screw (without angulation or compression). The gold (compression) drill guide will produce a drill hole that is offset from the center of the plate hole. When the screw is applied, this will apply compression to the fracture site. The gold (compression) drill guide has a small arrow on its surface, and this arrow should be pointing in the direction that compression is to be applied. The universal drill guide will allow the clinician to angle the screw within the screw hole. Further advances in plating technology have led to these plates being largely replaced by the limited contact dynamic compression plates (LC‐DCPs) (see below). Typical sizes available include 1.5, 2.0, 2.4, 2.7, 3.5, and 4.5 mm. Each size comes in a range of different lengths. The previously described DCP was further refined into the LC‐DCP (Figure 39.20). The underside of the LC‐DCP is scalloped rather than solid (as for the DCP). This modification addresses two primary issues with the DCP plating system. First, the scalloping of the undersurface of the plate reduces the area of the plate in direct contact with the bone, and this is thought to improve the vascularity of the area compared with the DCP system.12 In addition, the scalloping of the plate reduces the stiffness of the solid section of the plate, making it more closely match the stiffness of the section with holes. Since the stiffness is more closely matched, stress is not concentrated at the screw holes, as it is for the DCP system. The scalloping also has the added benefit of allowing any contour introduced to the plate with bending to be more evenly distributed along the plate, rather than concentrated at the screw holes as it is for the DCP system. Figure 39.20 Synthes 2.4 mm limited contact dynamic compression plate. (a) The superficial surface with oval compression holes. (b) A side view with the sloped compression holes. (c) The deep surface, which is in contact with the bone. The scalloped edges of this surface decrease the area in contact with the bone and have multiple benefits as described in the text. Source: © Rebecca Webb. As discussed, the scalloping of the undersurface of the plate has many benefits; however, it is important to note that the reduction in stiffness by scalloping reduces the strength of the plate when compared with the size equivalent DCP.13 Since the optimal strength and stiffness of a plate are currently unknown, it is unclear if this decrease in strength is of any clinical significance. In addition to the scalloping, the underside of the plate hole of the LC‐DCP plate system has been enlarged compared with the size equivalent DCP, and this allows the screw to be angled to a greater degree in the longitudinal plane (up to 40° for the LC‐DCP) than the DCP system (25° of longitudinal angulation). As for the DCP system, the LC‐DCP system allows screws to be inserted in neutral, in compression, and in an angled fashion, and it has similar drill guides. The LC‐DCP plating systems come in a range of sizes including 1.5/2.0, 2.0/2.4, 2.7, 3.5, and 4.5 mm. They are available in a range of lengths and available in titanium and stainless steel. The veterinary cuttable plate comes in two sizes: 1.5 mm (which can accept 1.5 and 2.0 mm screws) and 2.0 mm (which can accept 2.0, 2.4, and 2.7 mm screws) (Figure 39.21). Each plate is 50 holes in length and can be cut to size depending on the length that is required. These plates have round holes, which do not allow for compression at the fracture site as discussed above. The number of holes per unit length of the plate is significantly higher than that of the LC‐DCP and DCP plates. Given that it is a cuttable plate, the stiffness and strength of the plate are quite low. This can be increased by stacking a second plate on top of the first.14,15 Given the low strength of this plate, it is best suited to reconstructed fractures where there is load‐sharing between the bone and plate or in immature patients where rapid healing is expected. Figure 39.21 A juvenile toy breed canine patient with bilateral radius and ulna fractures. A craniomedial approach has been performed to expose both fracture sites, and the radial fractures have been plated with 2.0 mm veterinary cuttable plates with two 2.0 mm cortical screws proximally and distally on either side. Source: © Rebecca Webb. As discussed previously (see “Locking screws”), locking plates provide an “angle‐stable” construct between the screw, plate, and bone. As the screw is locked into the plate at a specified angle, these plates function more closely to external fixators than nonlocking plates. Due to this, they have also been named “internal fixators.” When compared with external fixators, these plates are much closer to the bone, increasing their mechanical strength. There are both fixed angle constructs, where the screw is locked into the plate at an angle specified by the manufacturer, as well as variable angle constructs, which allow a small amount of variation in the angle of the screw to the plate. Stability in both constructs is provided by the locking mechanism between the screw and the plate, which can be different for different systems. Due to the locking nature, the plate does not rely on direct bone contact for strength as the nonlocking plates do. This means that accurate plate contouring is less crucial for these systems. The reduction in contact between the bone and the plate may also improve the underlying blood supply and reduce bone resorption under the plate.16 In addition, the locking nature of the screws prevents bone fragment displacement during placement. Overall, locking plates give greater stability than standard, nonlocking plates.9,17 Due to the above improvements on the nonlocking plates, locking plates are more suited for bridging (see previously) and biological osteosynthesis where there is limited load‐sharing between the bone and plate. In contrast to more traditional plating techniques, which typically prioritize reconstruction of the fracture site for load‐sharing, biological osteosynthesis seeks to enhance healing by maintaining the biologic properties of the fracture. The biological properties are maintained by minimizing surgical intervention at the fracture site and not attempting anatomical reconstruction of the fracture. The goal of biological osteosynthesis is to achieve relative stability of the fracture site and secondary bone healing. This method of osteosynthesis has advanced over time and now includes the minimally invasive application of locking plates to adhere to the principles of biological osteosynthesis more closely.18 The angle‐stable nature of the implants increases the strength of any monocortical implants when compared with nonlocking systems. This makes them helpful for the reconstruction of articular fractures, such as the acetabulum, carpus, and tarsus if a screw is inadvertently aimed at the joint surface. They are also beneficial when performing “double‐plating” (i.e., orthogonal plating) and when using a plate as part of a plate‐rod construct, as some screws may need to be monocortical to avoid interference with the additional implants. To decrease the plate strain and likelihood of plate failure, recommendations have been suggested for the use of locking plates in human medicine. These include spanning a long section of bone (greater than three times the length of the fractured segment), limiting the screw‐to‐hole ratio to less than 0.5 and limiting the distance between the plate and bone to less than or equal to 2 mm.19,20 Locking plate constructs can be made stiffer by selecting a larger sized plate, adding additional implants (an IM pin or second plate), or by shortening the “working length” of the plate (i.e., by placing screws closer to the fracture site).10,21 Two screws per fracture segment are the minimum required when using locking systems. There is a minimal advantage to placing more than three screws per segment and placing the third screw toward the fracture site increases stiffness.22 The locking compression plate (LCP; Synthes) is a locking plate made from 316L stainless steel (Figure 39.22a), and it comes in a range of sizes, including 1.5, 2.0, 2.4, 2.7, 3.5, 4.5, and 5.5 mm, each with a range of lengths and shapes. The LCP plate has a combination hole or “combi hole” (Figure 39.22b), which can accommodate either a conventional nonlocking screw or a locking head screw. The end that accepts a nonlocking screw is a compression hole, which allows the clinician to apply compression to the fracture site. The locking end of the hole is threaded. The threads on this hole accept the threads of the locking head screw to create a locking mechanism. Given the combi hole allows both compression and locking to be applied to the fracture, the LCP plate can be used as a compression plate, as a locking plate, or using components of both features. The LCP plate also has a scalloped underside, like the LC‐DCP plate, with the same advantages as such. There are two drill guides for each size. For the locking screws, a locking drill guide is used. This guide is threaded and screwed into the threads of the plate at a fixed angle. In this way, the screw hole is drilled so that the angle is appropriate to allow the threads of the screw and plate to match and lock together. The other guide is a universal guide that can be used to apply a nonlocking screw in either compression or neutral modes. Figure 39.22 (a) A 2.4 mm locking compression plate (LCP®; Synthes). (b) A close‐up image of the holes of the plate in image (a), showing the combination plate hole, or “combi” hole. The hole has a compression end (to the left side of the plate hole) and a locking end (to the right side of the plate hole). Source: © Rebecca Webb. The String of Pearls plate or SOP™ plate is unusual in comparison to the other locking systems discussed here, as it uses standard cortical nonlocking screws. They are available in 2.0, 2.7, and 3.5 mm sizes in 316L stainless steel and titanium and can be cut to length (Figure 39.23). The plate consists of spherical “pearls,” which accept cortical nonlocking screws. The pearls are connected to each other with cylindrical sections or “internodes.” The screw head press fits within the “pearl” using a thread at the base of the screw hole as well as a contact ridge within the screw hole. The plate can be contoured in multiple planes, including medial to lateral bending, cranial to caudal bending, and torsion at the internode. To prevent the pearls from becoming deformed during the bending and twisting, bending tees are placed within the holes of the 2.7 and 3.5 mm plates (Figure 39.24). These plates can withstand a high degree of contouring, giving them an advantage over other plating systems (Figure 39.25). However, these plates can be bulky in comparison to a similarly sized LCP plate, so their use is limited in regions with minimal soft tissue coverage. Figure 39.23 A 3.5 mm String of Pearls (SOP™; Orthomed) plate in 316L stainless steel. Images show the top (a) and side views (b) of the plate without screws. The spherical “pearls” accept cortical nonlocking screws. The cylindrical “internodes” connect between the pearls and can be contoured in six degrees of freedom. Source: © Rebecca Webb. Figure 39.24 A 3.5 mm String of Pearls (SOP™; Orthomed) plate in 316L stainless steel. The bending irons have been applied across two pearls of the plate. The two pearls of the plate which are going to be bent have been filled with bending tees to prevent deformation of the plate holes during bending. Source: © Rebecca Webb. The FIXIN® system is a locking system that comes in three size ranges. The Micro Series (1.3–1.7 mm, developed for toy breed dogs and cats weighing 3–4 kg), the Mini Series (1.9–2.5 mm, developed for small dogs and cats weighing up to 10 kg), and the Large Series (3.0–3.5–4.0 mm, developed for large dogs weighing 15–70 kg). The plates are constructed from 316L stainless steel, and the screws are a titanium alloy and come in a range of straight plates and contoured plates. While historically there has been concern for galvanic corrosion when mixing two different types of materials for implants, this is suspected to not be a clinical problem when mixing titanium and stainless steel.23 The FIXIN system uses a threaded bushing within the screw hole and a conical taper to the screw head to lock the screw into the plate (Figure 39.26). In this system, the bushing screws into the plate and acts as an intermediary between the screw and the plate. Locking is achieved between the screw head and the bushing. The advantage of this system is the ease of removal if required. In other locking mechanisms, if the screw has been cold welded to the plate or if the screw head is unable to engage with the screwdriver, removal can be challenging. In comparison, the FIXIN® can be removed more easily, as the bushing is able to be easily removed. The addition of the bushing also increases the screw’s resistance to shear forces and allows for a thinner plate design. This allows more elasticity, promotes early callus formation, and can be easily used in regions with limited soft tissue coverage. The plates also come with K‐wire holes, which can be helpful for temporary fixation of the plate while screws are drilled. There are currently two locking plate systems which feature variable angle locking screws. In both systems, the locking mechanism can be maintained with a slight angulation to the screws. The ability to angle these screws is beneficial for complex fractures where screws need to be angled away from joint surfaces, other implants, or fracture lines. In traditional locking plate systems, the angulation of placement would necessitate the use of a cortical screw, which isn’t as strong. It is less ideal to use a cortical screw in these situations if there isn’t appropriate contact between the plate and the bone at the required screw hole location. The ability to angle a locking screw slightly while still maintaining the locking mechanism with the below systems can be very useful, particularly in these scenarios. Figure 39.25 (a) Pre‐operative lateral radiograph of a long oblique distal femoral fracture in a canine patient. (b) Postoperative lateral radiograph of a long oblique femoral fracture postfixation with a 9/64″ IM pin and a lateral 3.5 mm SOP™ plate (Orthomed) with six screws placed distally and five proximally. Source: Courtesy of Dr. Desmond Tan. Figure 39.26 (a) FIXIN® 3.0–3.5–4.0 mm series L support long plate with a 3.5 mm autolocking screw, note the blue‐colored bushings in the plate holes. (b) The FIXIN® plate from image (a) with the autolocking screw inserted into the distal most screw hole. Source: Courtesy of Christine A. Valdez. The Arthrex® OrthoLine™ system comes in a range of sizes, including 1.6, 1.6 broad, 2.0, 2.4, 3.0, 3.5, and 3.5 broad/4.0 mm. The plates in the 1.6–3.0 mm range are made of titanium, and the larger plates are made of 316L stainless steel. The plates come in a range of straight plates (Figures 39.19 and 39.27), T‐plates, and TPLO plates, as well as distal humeral condyle plates (Figure 39.28). The straight plates are designed to have a higher screw density toward the ends of the plate and have a central bridge within the middle of the plate with fewer holes to help span transverse and short oblique fractures; this means that a screw hole is less likely to be left open over the fracture line. The plates feature compression, slide, and locking holes. The plates were designed to allow multiple methods of temporary K‐wire fixation to the bone. As discussed for the FIXIN® system, it can be very helpful to temporarily stabilize the plate to the bone with K‐wires while the screw holes are drilled. In the OrthoLine™ system, the K‐wires can be placed within the K‐wire holes of the plate, through a bending plug, which fits within one of the locking screw holes, or using a Bb‐Tak (Arthrex®), which is a specific K‐wire with a small cylinder attached that can be drilled into the screw holes. Like the LCP and LC‐DCP™ plates, the underside of the OrthoLine™ plates is scalloped on the underside to limit contact with the bone surface, which is thought to improve periosteal blood supply. The plates can accept cortical, standard locking, and variable locking (titanium sizes only) screws. The standard locking screw is applied using a standard locking guide that screws into the threads of the locking screw hole, similar to the LCP (Synthes) system. The standard locking screws are angled at a fixed angle from the plate. The variable angle locking screws are available for the titanium plates only and allow the clinician to place them with up to 12° of angulation in any direction. To place the variable angle screw, a conical drill guide is used, which allows the clinician to angle the drill bit within a fixed 12° range. The system also comes with a 4.0 mm locking screw, which can be used in the 3.5‐mm and 3.5‐broad plates. Due to the larger core diameter of the 4.0 mm screw, this size further increases the strength of the construct for large and giant dog breeds. The T‐plates within this system have been designed with the locking screws angled away from the joint surface by 7.5° to enable safer placement close to joint lines. Figure 39.27 A 3.5 mm stainless steel broad 8‐hole Arthrex® OrthoLine™ plate. Note the small K‐wire holes for temporary fixation. Source: © Rebecca Webb. Figure 39.28 A 3.0 mm titanium right‐sided distal humeral condylar plate. The plate is pre‐contoured so that it fits the distal humeral condyle. The screw holes of this plate accept conventional locking, variable locking, and cortical screws. Note the small K‐wire holes for temporary fixation. Source: © Rebecca Webb. The polyaxial locking plate system (PAX®) is a titanium plating system that comes in sizes 2.0, 2.4, 2.7, and 3.5 mm. The plates come in reconstruction plates, lengthening plates (with a central region without screw holes), T‐ and L‐plates. The screws can be angled up to 10° from vertical. The PAX® system functions by using different titanium alloys for the screw and the plate. The alloy used for the screw head is approximately twice as strong as that of the plate. This allows the screw to cut its own threads into the plate as it is inserted, at angles of up to 10° from vertical. Generating adequate torque when inserting these screws is important to ensure that the variable locking mechanism functions appropriately. ILNs are used primarily in long bone fractures of the humerus, femur, and tibia, and occasionally, in the ulna of very large dogs. As with IM pins, their use is contraindicated in the radius. They consist of an intramedullary nail, which comes in a range of sizes (3, 4, 5, 6, 7, 8, and 10 mm in diameter), each with a range of lengths (Figure 39.29). These nails typically have two holes on either end, which accept either screws or bolts. These screws or bolts affix the intramedullary nail to the cortical bone of the proximal and distal fragments and give stability to the construct (Figure 39.30). Figure 39.29 BioMedtrix® I‐Loc® interlocking nails in 3, 4, and 5 mm widths of various lengths. Source: Courtesy of Christine A. Valdez. Figure 39.30 (a,b) Pre‐operative lateral and craniocaudal radiographs of a canine patient with a left comminuted tibial diaphyseal fracture. (c,d) Immediate postoperative lateral and craniocaudal radiographs of the same patient postplacement of a 172 mm long, 6 mm I‐Loc® interlocking nail (Biomedtrix®) with two bolts proximally and distally. (e,f) Six‐week postoperative lateral and craniocaudal radiographs of the same patient showing a marked progression in healing callus. Source: Courtesy of Dr. Desmond Tan. ILNs resist all forces placed upon a fracture. The intramedullary nail resists bending and compression, and the fixation bolts provide axial and rotational support. ILNs have some biomechanical advantages over conventional bone plates. As they are placed near the neutral axis of the bone, they tend to be subjected to more compressive forces during ambulation.24–27 In comparison, bone plates are placed eccentrically from this axis and are subjected to more bending forces.28,29 The bending forces that plates experience can predispose them to fatigue failure at lower loads compared with ILN, especially in fractures with large, comminuted segments.30,31 In addition, the intramedullary placement of the ILN prevents it from being subjected to screw pull‐out, which can be seen in plates, particularly when placed in weak bone. There are multiple ILN systems, which use either screws or bolts to stabilize the fragments. While these implants primarily provide axial and rotational support to the fragment, earlier implant systems using screws would fail secondary to the bending of the screw. Due to their larger core diameter, bolts are more resistant to bending forces compared with screws, and their use is preferred to reduce the risk of implant failure. This chapter will focus primarily on the I‐Loc® IM Fixator system (BioMedtrix®) (Figure 39.31). This system uses threaded tapered bolts that screw into threaded cannulations in the nail. As discussed previously with the other implant systems, there are regular continuing education courses that clinicians can pursue for more in‐depth knowledge of and training for the system they will be using. These courses are essential for achieving successful clinical results with the ILN. Figure 39.31 Instrument set for a 3 mm I‐Loc® system. (a) General I‐Loc® instruments. (b) Instruments specific to the 3 mm size I‐Loc® system. Source: Courtesy of Christine A. Valdez. The ILN is typically used in diaphyseal fractures of the bones listed previously, provided there is enough cortical bone in the proximal and distal fragments to accept two fixation screws or bolts. The screws or bolts should be placed a length of at least 1–2x the bone diameter away from the fracture site to prevent the development of fissures.32,33 The clinician should pay particular attention to the presence of any fissures on the pre‐operative radiographs, which may preclude ILN placement. The proper use and application of ILN involves selecting a nail of appropriate size and length, drilling a hole in the bone to allow the nail to be passed into the medullary canal, and locking the nail in place with screws or locking bolts. The ILN can be placed either in an open approach or in a minimally invasive manner with the use of fluoroscopy. The use of fluoroscopy and minimally invasive placement of the ILN does not disturb the fracture site and leads to improved fracture site biology, promoting early healing for these patients. Based on calibrated pre‐operative radiographs, the width of the medullary canal at its narrowest point (i.e., the isthmus) is measured. This is easiest to perform on the contralateral (noninjured) limb. The width of the nail chosen should be the largest nail without exceeding 70–90% of the isthmus, which reduces the risk of iatrogenic fracture during insertion.24,25 To limit stress‐shielding with the I‐Loc (BioMedtrix), a limit of 75% of the isthmus is suggested.34 Typically, nails 3–4 mm in diameter are appropriate for use in cats and small dogs (5–15 kg), the 6 mm nail is appropriate for mid‐sized dogs (15–30 kg), the 7 mm nail is usually appropriate for large dogs (up to 40 kg), and 8–10 mm nails are usually used for giant breed dogs (>40 kg).9 The length of the nail should span most of the length of the bone. Similar to the selection of the width of the nail, this is most easily measured from radiographs of the nonfractured contralateral limb. The ILN is applied in a normograde manner to allow the use of an aiming guide for placement. The first step in application is gaining entry to the medullary canal of the fractured bone. This is typically performed using a Steinman pin. Following this, the medullary cavity is prepared to allow the ILN to pass within it. The cavity must be prepared to the appropriate width and length for the selected nail, and this is typically performed with a series of dedicated awls. The ILN to be inserted is attached to an extension piece, which is then attached to a handle. The handle is used to insert the ILN. Once the ILN is inserted, an aiming jig is attached to the handle to align the drilling of the locking bolts with the holes of the ILN (Figure 39.32). It is of great importance at this point in the fixation to accurately assess the alignment of the joints proximal and distal to the fracture fragment. While anatomic reconstruction of the fracture fragments is not necessary for this technique to be successful, it is of vital importance that the joints are accurately aligned, or an angular limb deformity will result. For each locking bolt, there will be two drill bits to be used to place it. There will be a drill bit for the cis‐cortex (i.e., near) and a separate drill bit for the trans‐cortex (i.e., far). Once the cis‐cortex has been drilled, a feeler handle is used to palpate within the medullary canal for the threads of the ILN within. This helps the clinician to confirm that the drilling has accurately targeted the hole of the ILN within. Careful drilling and assessment of these holes are very important to ensure that the bolt is passing through the ILN hole. Figure 39.32 The handle (horizontal piece) is connected to an aiming jig (vertical piece). The aiming jig has measurements on it, which correspond to the locations of the ILN holes for different ILN lengths. In the clinical case, the interlocking nail would be attached to the handle on the left‐hand side of the image via an extension piece. Source: Courtesy of Christine A. Valdez. Prior to drilling, it is important to tighten the connections between the aiming jig and the nail to ensure they are not loose, as this can potentially lead to missing the ILN hole during drilling. Using a sharp drill bit can also be helpful to maintain the trajectory of the aiming jig. Following this, the trans‐cortex is drilled, and the hole is filled with an alignment post. The remainder of the holes are drilled in the same manner. Once the holes have been drilled, one alignment post is removed at a time, and a specialized depth gauge is used, which gives a reading for both the cis‐ and trans‐cortical lengths for the bolt. A bolt is then cut to length on both the cis‐ and trans‐ends and applied to the ILN. The remainder of the bolts are placed sequentially, usually proximally to distally. Finally, the handle and extension piece are removed from the ILN. ESFs are external devices that are attached to the bone through percutaneously placed implants to stabilize fractures, to temporarily immobilize a joint, or to correct bone deformities. ESF consists of fixation pins or wires (Figure 39.33), connecting clamps (Figure 39.34), and connecting bars (Figure 39.35). ESFs are commonly used in open fractures, fractures with soft tissue damage, or in comminuted fractures. ESF has some unique advantages compared with conventional plating. They can be applied in a closed, or open but do not touch manner, making them helpful for biological osteosynthesis.35–37 By avoiding an approach to the fracture site or performing minimal disruptions of the fracture site at the time of surgery, the local soft tissues and vasculature can be preserved. In addition, the implants make minimal contact with the periosteum, further limiting damage to the vascular supply of the fracture. The implants aim to align the joint surfaces and adequately stabilize the fracture fragments to enable secondary bone healing to occur. This may speed up overall healing compared with more invasive internal fixation methods.35,37,38 Figure 39.33 Fixation pins and wire. (a) 1.6 mm Stopper Fixation Wire (IMEX®). This wire features a special cutting point that increases accuracy in placement. The bead on the wire contacts the bone surface when placed to prevent migration of the bone along a smooth wire. (b) Top: 2.5 mm Duraface Fixation Half‐Pin, NP (No‐point) (IMEX®); bottom: 2.5 mm Centerface Fixation Full‐Pin (IMEX®). Both implants are fixation pins. The threaded sections are placed within the bone, then the smooth sections are secured with a connecting clamp. Source: © Rebecca Webb. Figure 39.34 (a) Small SK® ESF Clamp, Single (IMEX®). The pin‐gripping bolt is visualized on the right. Through this, the fixation pin is secured. Centrally, the open hole is designed to fit the connecting bar. The bolts on the left side of the image are used to secure and tighten the gripping bolt (top) and the connecting bar (bottom). (b) Small SK® ESF Double Clamp (IMEX®), the double clamp is required for attaching two connecting bars to each other (one through each of the central holes). Source: © Rebecca Webb. Figure 39.35 (a) Connecting bars come in a range of different lengths and are made in different materials. All connecting bars in the image are 6.3 mm in diameter and are designed for the Small SK® ESF system (IMEX®). The two connecting bars on the left are made of titanium and the two on the right are carbon fiber rods. Each are shown in 100 and 150 mm lengths. (b) A 6.3 mm diameter titanium connecting bar with a Small SK® ESF Single Clamp attached (IMEX®). The gripping bolt, which holds the fixation pin, is visualized on the right of the image. Source: © Rebecca Webb. The external position of the implants makes them very useful for stabilizing open fractures associated with large wounds or contamination of the fracture site. In these cases, access to the wounds and contaminated regions is maintained, so the wounds can be adequately treated while the fracture heals. In addition, for cases with heavy contamination, the use of an external frame, which is eventually removed, is helpful when compared with internal implants. With internal implants, there is a potential for biofilm formation in infected regions, often necessitating future surgical implant removal. However, with the ESF, implants are typically removed easily under sedation when healing is appropriate. Another advantage of ESF is that the rigidity of the ESF system can also be altered (typically decreased throughout the healing process). As a fracture callus forms and starts providing some stability to the fracture site, the initial level of rigidity is no longer necessary. Individual components can then be gradually removed, in a process called dynamization, to approximate the physiologic needs of the fracture site more closely.39–41 There are several disadvantages to the ESF systems that should also be noted. First, closed application, although it is beneficial to preserve the biological environment of the fracture, is best performed with intra‐operative imaging, which is not always widely available. Alternatively, the clinician can use pre‐operative calibrated radiographs to measure and help approximate implant placement locations, use a limited open approach, or can use a sterile hypodermic needle to help delineate the bone edges in each region. Second, given that the fixation pins and wire are placed percutaneously, they can act as a tract for ascending bacterial contamination. Pin tract infections occur with some frequency and can lead to early pin loosening. Appropriate postoperative pin tract care is important to minimize this complication. They are also not ideal for every bone, such as the femur, which would involve pins traversing a large mass of muscle to reach the affected bone. When compared with conventional plates, the connecting bars of an ESF are eccentric to the central axis of the bone. This places them at a mechanical disadvantage when compared with bone plates, which can lead to pin loosening over time. If this pin loosening occurs before the fracture is healed, the construct will become weaker and may not be able to adequately stabilize the fracture for healing. Due to this, ESF systems are not designed for long‐term use or for use when delayed healing is anticipated. Lastly, the postoperative care is more intensive on the owner, patient, and veterinary staff for ESF. Regular bandage changes are required in the postoperative period to prevent pin tract infections, which may not be tolerated by some patients or clients. There is also a risk of the external frame injuring the patient or client at home. It is important to recognize the advantages and disadvantages of ESF when choosing a surgical stabilization method. There are several types of fixators available, including linear, circular, hybrid, and acrylic systems. For each of the systems, there are a range of brands. The proper use and application of ESF involve selecting pins or wires of appropriate size and strength for the patient, drilling holes in the bone to allow the pins or wires to pass through, and securing the pins or wires to the frame or external bar with proper tension. ESF can be used alone or in combination with other implants (such as intramedullary pins). Pins can be classified as either half‐ or full‐pins. Half‐pins penetrate the soft tissues on only one side, leaving one end exposed for incorporation into the ESF frame. In comparison, full‐pins penetrate the soft tissue on both sides of the limb and have pin ends exposed on both sides, which can be incorporated into the ESF frame. Anatomically safe corridors for pin placement have been described and should be referenced when placing fixation pins.44,45 Pins should be placed as centrally within the bone as possible to maximize stability and pull‐out resistance. The half‐pins should be placed so that the threads engage both cortices and the tip of the pin is protruding slightly into the soft tissue. If the pin is placed too far into the soft tissue, then the clinician should note that retracting the pin will degrade the quality of the engagement of the pin with the bone. Therefore, clinicians should pay close attention to appropriate initial placement. Classification of ESF frames can be helpful to document the surgical procedure performed, as well as to help predict the mechanical performance of the frame. The classification scheme initially classifies based on whether a construct is unilateral (has a connecting bar on one side only) or bilateral (connecting bars on both sides) (Figure 39.36). It then considers whether the frame is uniplanar (pins placed in a single plane) (Figure 39.37) or biplanar (pins placed in more than one distinctly different plane). This results in four different classification types, which are ranked in order of weakest to strongest (Figure 39.38). As the complexity of the frame increases, so does the stiffness and strength of the construct.46,47 However, the added strength should be balanced with the increase in biological compromise by the added pins. The anatomical safe corridors that were mentioned previously are very important when planning a construct. It is also important to note that there are very few locations where there are safe corridors on both sides of the limb, therefore, any full‐pin will invade into at least one unsafe corridor. For this reason, traditional type II frames (which use only full pins) are rarely used. Over time, ESF systems have been refined and the pins, clamps, and connecting bars improved, so that type Ia or type Ib frames now provide sufficient stability for bone healing. Due to this, the need for full pins and more complex frames is reduced.48 Figure 39.36 An avian patient postoperative for a short oblique tibiotarsus fracture. The fracture has been stabilized with a type II ESF. Proximal is to the top of the image, distal is to the bottom of the image. Source: © Rebecca Webb. Figure 39.37 A juvenile canine patient with a type I ESF placed on the lateral aspect of the left ilium for a caudal ilial fracture. This is an uncommon use for the ESF system, which was chosen in this patient due to the young age of the patient and chronicity of the fracture. The head is to the left of the image. Source: © Rebecca Webb. The pin‐bone interface is the most common location of failure of an ESF. Failure at this location leads to pin loosening, which may prevent healing or may require removal of the frame prior to bone healing. This could result in additional surgery to stabilize the limb. Due to this, several techniques have been devised to help maintain this interface, and therefore, extend the longevity of the ESF frame. Figure 39.38 Four different classification types listed in order of weakest to strongest. Tips and techniques used to preserve the pin‐bone interface include: Generally, three to four pins are placed per bone segment on either side of the fracture. The stiffness of the fixation can be increased by increasing the number of pins placed; however, the additional stiffness achieved in placing more than four pins per fragment does not offset the increase in morbidity of placing more pins. The first pins placed are the two that are farthest from the fracture site. Once these are placed, a connecting bar is added, and the alignment and reduction are altered as needed. Once the alignment and reduction are adequate, the pins closest to the fracture site are placed to maintain the alignment. Following this, additional pins are placed between the two pins on either side of the fracture to increase the stiffness of the construct. Generally, pins are placed half a bone diameter from the fracture site and ¾ of a bone diameter from the adjacent joints to obtain maximal bone purchase and avoid joint penetration. Acrylic ESF is ESF where the clamps and connecting bars have been replaced by an acrylic (typically methyl methacrylate or epoxy resin) column (Figure 39.39). These are also called free‐form skeletal fixators, as the construct is custom‐molded for the patient. Different‐sized pins can be used in the fixator, and the shape of the connecting bar does not have to be linear. The acrylic columns have the benefits of being strong, lightweight, and economical. Due to their lightweight nature, their use is common in small breeds and exotics. The significant drawback of the acrylic fixator is the inability to alter the fixation postcuring of the acrylic. This also limits the staged disassembly of the construct as previously described. In acrylic fixators, disassembly can be achieved by cutting a pin or cutting the connecting bar between pins of a fracture segment to decrease stability.51 The strength of the acrylic construct can be increased by increasing the size of the acrylic column.52 The effect that bending the fixation pins and incorporating them within the acrylic (like rebar) has on the strength of the construct has not been studied; however, it is thought to help increase pin purchase within the acrylic and may help to prevent pin pull‐out. Figure 39.39 A type I acrylic frame on an avian femoral fracture. Not visualized is an intramedullary pin, which has been incorporated into the acrylic frame to increase construct strength for this patient. Proximal is to the top of the image, distal is to the bottom of the image. Source: © Rebecca Webb. A circular external skeletal fixation (CESF) frame uses rings and tensioned wires instead of larger bone pins to secure the bone fragments. The wires pass through the bone and then are secured to a ring on either side with bolts instead of clamps (Figure 39.40). Rings are placed around the proximal and distal fragments and are then connected with connecting rods to create the final construct. Circular components are particularly useful with small juxta‐articular fragments due to their ability to use wires instead of pins for fixation. Wires allow axial micromotion to occur during the loading of a fracture. This micromotion is limited and has been shown to stimulate osteoblasts and bone healing.53 The appropriate amount of wire tension is crucial for this effect to occur, and a tensioning device is recommended to achieve it. Figure 39.40 Intra‐operative photograph of a patient with an antebrachial angular limb deformity. A Stretch Ring has been placed in line with the joints proximally and distally and secured with two Stopper fixation wires (IMEX®) in each section. Once a closing wedge resection is performed to correct the deformity of the radius, connecting bars will be attached to the rings, the limb straightened, and the circular ESF will be used as a form of temporary stabilization prior to plate placement. Distal is to the top of the image, proximal is to the bottom of the image. Source: © Rebecca Webb. Figure 39.41 A range of circular ESF components. The ring on the left is a Stretch Ring. The two rings on the right are two different sizes of Full Rings (50 and 84 mm) (IMEX®). Source: © Rebecca Webb. Figure 39.42 A wire tensioner is used to appropriately tension wires in the circular section of the ESF (IMEX®). Source: © Rebecca Webb. Figure 39.43 A slotted wire fixation bolt (IMEX®) attached to a circular ring. Wires can be passed through the bolt in two locations, through the central cannula of the bolt or through the slot on the underside of the head of the bolt (visualized on the left side of the bolt in the image). Source: © Rebecca Webb. Figure 39.44 A half‐pin fixation bolt can also be applied to the ring to allow fixation pins to be secured to the ring instead of a wire. The fixation pin is passed through the central cannula of the bolt (IMEX®). Source: © Rebecca Webb. Hybrid external fixators are fixators with a circular component on one end of the fracture and a linear component on the other end of the fracture (Figure 39.46). These fixators are most commonly used when there is a small juxta‐articular fragment, and the circular component is used to gain adequate fixation of the small fragment. The hybrid ESF then takes advantage of the ease of application and preservation of safe corridors of the linear system for the other, larger bone fragment. Biomechanically, they exhibit characteristics of both the static axial stiffness of the linear ESF and the dynamic micromotion of the circular system.59 Using a similar classification system as for the linear ESF, the connecting bars can be placed in a type IA (unilateral, uniplanar), a type IB (unilateral, biplanar), or a type II (bilateral, uniplanar) configuration. Figure 39.45 The VariBall Locking Hybrid Connecting Rod (IMEX®). The multi‐directional locking ball is integrated with a 6.3 mm carbon fiber rod. Source: © Rebecca Webb. Figure 39.46 (a) Pre‐operative craniocaudal radiograph of a canine patient with a distal tibial comminuted open fracture. (b) Postoperative craniocaudal radiograph of the same patient postsurgical repair with a hybrid I‐B small SK ESF frame (IMEX®). (c) Postsurgical repair of the right tibial fracture with a hybrid I‐B small SK ESF frame (IMEX®) showing the components. Following confirmation of appropriate fixation on postoperative radiographs, the pins were then cut to the level of the clamp, and the wires were bent to prevent injury and cut to the level of the ring. Source: © Rebecca Webb. Stabilization of fractures with highly stiff constructs can lead to a delay in bone healing. In some cases, such as very comminuted fractures with minimal load‐sharing, a very stiff construct is required in the early stages of fracture healing. However, as a fracture callus forms, the level of stability that the fracture requires is decreased, and continued stabilization with a stiff frame at this point can actually slow bone healing. In these cases, staged, partial disassembly can be performed to destabilize the construct and help improve bone healing. During the healing process, regular radiographs should be taken, which will help guide when destabilization is appropriate. This should be coupled with palpation of the fracture site for stability with the frame slightly loosened. There must be sufficient stability present at the fracture site to tolerate the destabilization, or destabilization could lead to delayed union or implant failure. Generally, the timing for destabilization is 4–6 weeks following placement for young dogs, 6 weeks following placement for adult dogs, and 8–10 weeks following original ESF placement for older dogs and cats.40 There are multiple options for destabilization, including pin removal (pins that are causing patient morbidity [discharge, infection, or loosening] should be prioritized for this), removal of the “near” pins (those closest to the fracture site), removal of one connecting bar and associated pins (in a type Ib and higher construct), downsizing the clamps, removing augmentations (tie‐in IM pins or diagonal connecting bars) and connecting bars, or replacing aluminum or titanium connecting bars with carbon fiber.
39
Orthopedic Implants
Introduction
Steinmann Pins/Kirschner Wires
Intramedullary Pin (IM Pin)
Small Fragment Fixation
The ends of the pins should be bent to prevent migration and cut short. There are multiple ways to achieve a bend in a K‐wire, including commercially available pin benders, a metal Frazier suction tip, Jacobs chuck, or needle drivers (Figure 39.6). Most commonly, a “Z‐bend” technique is utilized, which uses two bends to achieve an acute angle. To perform the bend, the bending instrument is applied over the K‐wire as close as possible to its insertion on the bone, and the K‐wire is bent as acutely as the instrument will allow. The bending instrument is then withdrawn slightly further up the K‐wire, and a second bend is performed in the opposite direction (to form the “Z”). Most commonly, this second bend is created by the surgeon pushing the instrument toward the floor and medially or laterally away from the bone (Video 39.1; Figure 39.7).
The K‐wire placed in Video 39.1 was subsequently removed from the bone to demonstrate the “Z‐bend” within the wire. The wire at the bottom of the image was placed within the bone, the two bends performed to create an acute bend on the K‐wire are shown in the remaining pin.
Cross‐Pinning
Orthopedic Wire
Methods of Securing Orthopedic Wire
Cerclage
Tension Band
Bone Plates and Screws
Screws
Cortical Screws
Cancellous Screws
Locking Screws
Cannulated Screws
Headless Compression Screws
Screw Placement
Screw Uses
Lag Screw
Positional Screw
Plate Screw
Plates
Functions
Neutralization Plate
Bridging Plate
Buttress Plate
Compression Plate
Nonlocking Plates
Dynamic Compression Plate (DCP)
Limited Contact Dynamic Compression Plate (LC‐DCP)
Veterinary Cuttable Plate (VCP)
Locking Plates
Fixed Angle Locking Plates
Locking Compression Plate (LCP; Synthes)
String of Pearls (SOP™; Orthomed)
FIXIN ® (Intrauma)
Variable Angle Locking Plates
OrthoLine™ System (Arthrex® Vet Systems)
PAX® System (Securos Surgical®)
Interlocking Nail (ILN)
Implant Selection
Application
External Skeletal Fixation (ESF)
Linear External Skeletal Fixation
Components
Classification
Pin‐Bone Interface
ESF Placement
Acrylic External Skeletal Fixation
Circular External Skeletal Fixation
Components
Hybrid External Skeletal Fixation
Frame Destabilization and Removal
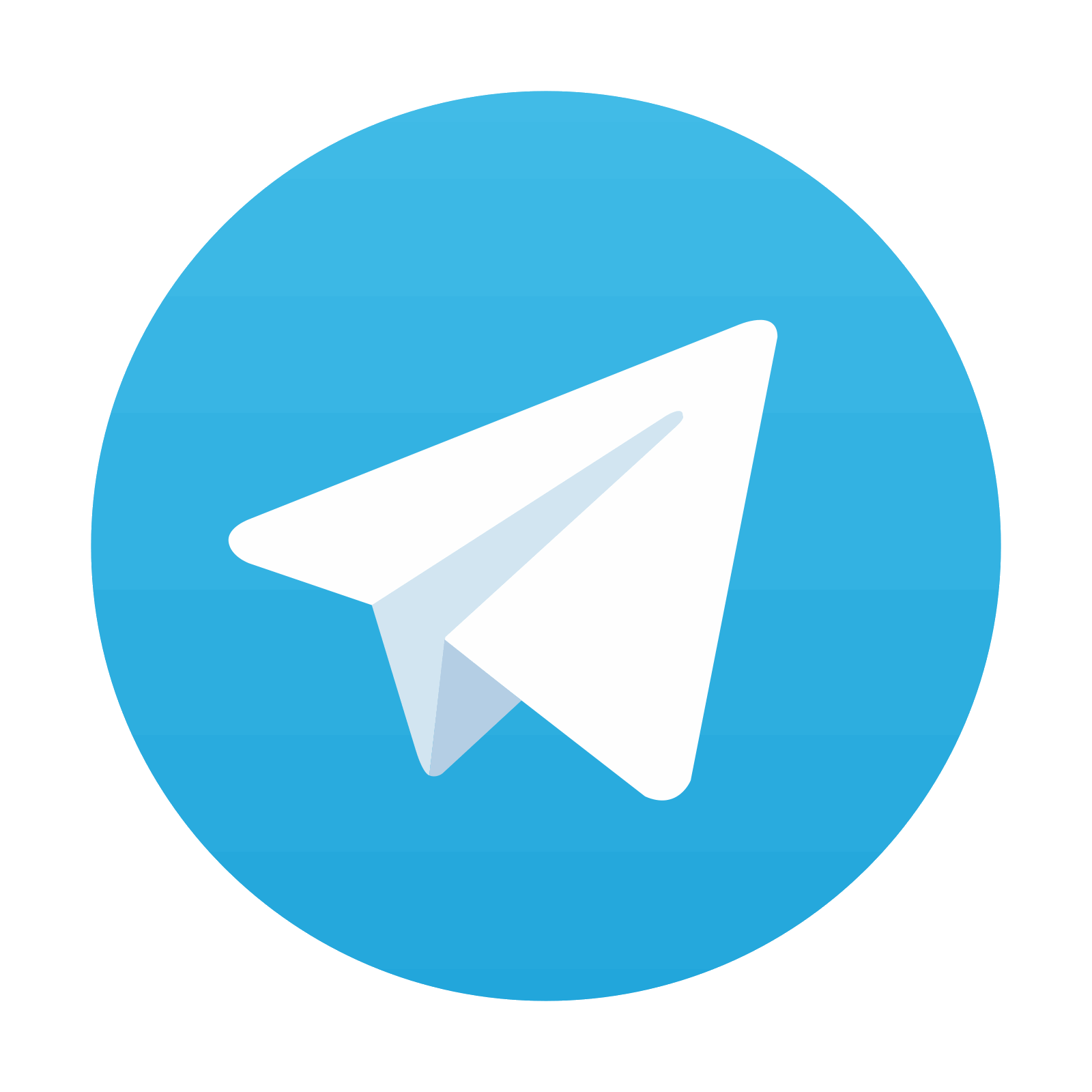
Stay updated, free articles. Join our Telegram channel
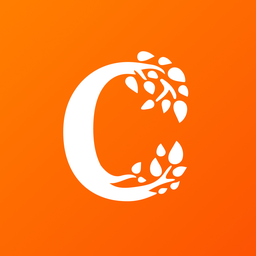
Full access? Get Clinical Tree
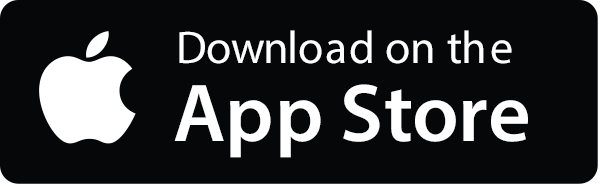
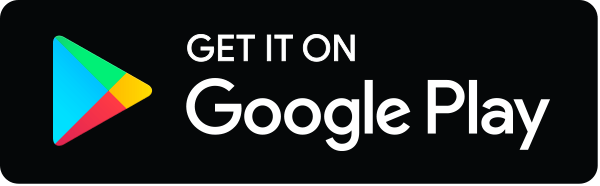