Bradley T. Simon1 and Ignacio Lizarraga2 1 Department of Small Animal Clinical Sciences, College of Veterinary Medicine and Biomedical Sciences, Texas A&M University, College Station, Texas, USA, and Veterinary Dentistry Specialists, Katy, Texas, USA 2 AgResearch Limited, Grasslands Research Centre, Palmerston North, New Zealand Opiates are drugs derived from opium, which is the latex of unripe seed pods of Papaver somniferum that contains naturally occurring alkaloids. Opioids are not naturally occurring drugs; rather, they are partially or completely synthesized in the laboratory. Both opiates and opioids interact with opioid receptors. In this chapter, the term “opioid” will be used to refer to naturally occurring or synthetic drugs that interact with opioid receptors. Opioids are the prototypical analgesics, antitussives, and antidiarrheal drug class. The opioid receptor system has been identified in a variety of amphibians, fish, reptiles, birds, and mammals [1,2]. Humans have used opium (from Papaver somniferum) for its analgesic effects for more than 8000 years [3]. The earliest documented use of opium in domestic animals, attributed to Robert Boyle, dates to the 17th century. Boyle focused on opium as a poison and wrote: “we have more than once given to a Dog, without much harming him, such a quantity of Opium, as would probably have suffic’d to have kill’d several Men” [4]. In 1659, in collaboration with Christopher Wren, Boyle injected an infusion of opium into a superficial pelvic limb vein causing deep stupor to an experimental dog [5]. In 1664, Robert Hooke proposed a practical union between opiate narcosis and surgery [4]. At that time, opium had been used in combination with alcohol and other ingredients to form various forms of laudanum, or tincture of opium. This became widely used to prepare patients for surgery until the discovery of effective anesthesia. Paregoric was a weaker preparation, named after the Greek word for “soothing” or “consoling” and included opium, honey, licorice root, camphor, aniseed, and wine [6]. Recognizing the limitations of opium for surgical anesthesia, James Moore reported in 1784 on opium’s postoperative analgesic effects [6]. In 1805, Friedrich Wilhelm Adam Sertürner reported the isolation of an alkaline substance from opium, which he later named “morphium” [7]. Sertürner administered an aqueous alcoholic extraction of the salt to four dogs and a mouse. The dogs vomited, convulsed, and appeared sleepy, but did not sleep; one dog died [8]. Although these early studies did not provide evidence of morphine’s analgesic effects, the isolation of morphine permitted accurate calculation of doses. The use of morphine became widespread following the development of the hypodermic needle and syringe in the 1850s. In 1908, Frederick Hobday reported that morphine’s effects varied considerably by animal species with cats and horses experiencing delirium and dogs experiencing narcosis, although a few displayed idiosyncratic reactions. He suggested a dose of 1/16th of a grain per pound of body weight to induce narcosis and analgesia in dogs [9]. In 1917, Howard Jay Milks reported that, other than in dogs, morphine had no value to relieve pain in veterinary animal species. However, he mentioned that two to five grains of morphine‐induced sedation in horses and one‐fifth to five grains had anesthetic‐sparing effects in dogs [10]. A limiting factor for the use of morphine in veterinary medicine was its excitatory effects. In 1921, Fröhner reported excitement combined with sensory and motor depression in horses administered morphine at doses of 0.4, 0.75, 1.5, and 10 g [11]. Although body weight was not reported, these morphine doses are considerably higher than those recommended in contemporary equine practice. In 1937, Amadon and Craigie described minimal analgesia and excitement in pain‐free horses administered morphine at doses of 0.2 and 0.5 mg/kg, respectively [12]. This may have prompted many to focus on the adverse effects rather than the analgesic actions of morphine and other opioids [13]. The increased use of opioids to provide analgesia in veterinary patients can be attributed to Yoxall who proposed that it was the veterinarian’s duty to alleviate pain whenever it may occur [14]. Since then, many opioid drugs in a variety of dosage forms and routes of administration have been used in animal species of veterinary interest. These have included the intra‐articular administration or intravenous regional limb perfusion of morphine to induce analgesia and prevent inflammation associated with orthopedic procedures in dogs and horses [15–19], the subcutaneous, intramuscular, intravenous, or intranasal administration of high‐concentration injectable buprenorphine solutions for analgesic purposes in cats and dogs [20,21], the transdermal administration of fentanyl contained in patches in dogs, pigs, and sheep [22–25], the subcutaneous administration of extended‐release buprenorphine solutions for analgesia in mice and rats [26,27], the transdermal administration of long‐acting fentanyl and buprenorphine solutions for analgesia in cats and dogs [28,29], and the buccal transmucosal administration of buprenorphine gels for analgesia in dogs [30]. The 1971 Convention of Psychotropic Substances, a United Nations treaty, introduced control provisions for narcotics. These provisions aimed to prevent diversion and abuse by limiting the prescription and clinical use of controlled substances to licensed parties. Global jurisdictions introduced systems and agencies to comply with these provisions. Moreover, under federal, state, and local law (where appropriate), controlled drugs must be maintained in locked storage areas with use documented in a log subject to inspection. In the United States (US), the Controlled Substances Act places regulated substances into one of five schedules (I to V). Scheduling is based on the substance’s medical use, potential for abuse, and safety or dependence liability. The abuse potential is a determinant in scheduling; Schedule I substances have a high potential for abuse and psychological and/or physical dependence, and Schedule V substances represent the least potential for abuse. In the 19th and the early 20th centuries, the US experienced an opiate crisis involving over‐the‐counter opium, morphine, and heroin products, and the spread of opium dens. Consumer advocacy and legislation ended the over‐the‐counter sale of opiate products and outlawed opium dens [31]. The contemporary North American opioid crisis, also known as the “opioid epidemic,” has been a decades‐long crisis with three major upsurges. The first upsurge started in 1995 and involved a profit‐driven increase in prescription opioids that were misleadingly marketed as safe and low‐risk extended‐release analgesics. The second upsurge began around 2010 when drug traffickers realized that people addicted to prescription opioids were a lucrative target for heroin. The third upsurge began around 2014 and has been characterized by illegal drug producers adding potent synthetic opioids to forged pharmaceutical pills, heroin, and stimulants [32]. Initial anecdotal evidence suggested [33], and more recent scientific evidence has confirmed [34–36], that veterinarians are a source for misused prescription opioids. An example of prescription opioid misuse is opioid diversion, which is the illegal distribution or abuse of a prescribed opioid or its use for purposes not intended by the prescriber. Some pet owners seek opioids from veterinarians for their own use and request opioids for their pets when they are not needed or recommended. Some have even intentionally made an animal ill, injured an animal, or made an animal seem ill or injured to obtain opioids [34]. Some may solicit opioid prescriptions from multiple veterinarians for the purpose of misuse, which is known as “vet shopping” [36,37]. In addition to clients, veterinary practice staff, including veterinarians, have been involved in opioid diversion [34,35]. Thus, opioid diversion could contribute to the growing opioid crisis, and veterinarians have a role in preventing opioid abuse and misuse. A few studies have shed some light on the situation veterinarians in the US face regarding opioid diversion and vet shopping. In a study conducted in 2018 involving 697 general veterinarians practicing across the US, 63.5% of veterinarians expressed concerns for opioid diversion to pet owners and 24.7% to staff within the clinic [35]. Mason et al. reported in 2018 that out of 189 veterinarians practicing in Colorado, 73% had either fair, poor, or no veterinary medical school training on opioid abuse or misuse and 64% had not completed continuing education on best practices for prescribing opioids since entering practice. Opioid abuse prevention (81%), pain management guidelines (55%), and how to engage electronic resources (54%) were identified as continuing educational priorities. Thirty‐six percent of respondents recommended improving prescription drug monitoring program (PDMP) guidelines and tutorials to help improve access and utilization [34]. A study including 14 veterinarians practicing in tri‐state Appalachian counties (Tennessee, n = 8; Kentucky, n =4; and Virginia, n = 2) found that 93% of respondents had heard of vet shopping, 57% had encountered vet shopping in their own practice, 86% indicated vet shopping was a problem in tri‐state Appalachian counties, and 71% had received no training on prevention and/or management of vet shopping. Respondents mentioned more efficient communication with other veterinarians and medical professionals including pharmacists, a valid veterinary–client–patient relationship (VCPR), more education for themselves and/or their staff, better tracking of animal prescriptions through databases, and judicious use of opioid prescriptions as means to prevent and control vet shopping [36]. Enhanced curricular offerings, continuing education trainings, and workplace policies, practices, procedures, and monitoring may foster opioid stewardship among veterinarians and may mitigate the risks of opioid diversion by both staff and clients. The implementation of mandatory PDMP enrollment for healthcare providers may deter opioid diversion and foster opioid stewardship [34,38]. As of April 30, 2021, and except for Missouri, all states and the District of Columbia had a PDMP in place. A total of 47 states had PDMP use mandates for any clinician before controlled substances may be prescribed. Of these, 37 states exempted veterinarians from mandates and 10 did not: Alaska, Connecticut, Indiana, Maine, Oklahoma, and West Virginia required veterinarians to check PDMP records of owners in some instances; and Arkansas, District of Columbia, Rhode Island, and South Carolina required veterinarians to check records of animals. None required veterinarians to check records of both owners and animals [37]. Although PDMP use mandates might improve controlled substances prescribing behaviors by veterinarians and deter opioid diversion, the benefits of these mandates should outweigh their costs and consider the impact of their implementation. For instance, time cost for veterinarians, incompatibility of PDMPs with veterinarians’ electronic medical record systems, privacy concerns among animal owners, lack of linkages between animal and owner PDMP records in many states, possibility of multiple PDMP records for animals, scope and types of information available to veterinarians during a PDMP review, protocols to detect and report suspected cases of veterinary shopping, and regulations for interstate sharing of PDMP data from animals and owners must be considered [37]. In line with this, the American Veterinary Medical Association has lobbied that PDMPs not be implemented for veterinarians until further evidence and better systems are put in place. Published information on the utility of PDMPs to identify potential vet shoppers is scarce. In a survey of 50 state PDMPs, Simpson concluded that less than 10 vet shoppers nationwide would be identified by PDMPs per year [39]. Using data collected between 2014 and 2019 from US retail and mail‐order pharmacies, the number of potential vet shoppers with prescriptions from greater than four veterinarians during a year for opioid cough‐and‐cold medications increased from 150 in 2014 to 1348 in 2019; for opioid analgesics, this number peaked at 868 in 2016 before decreasing to 733 in 2019 [37] (Table 23.1). The temporal distribution, use, prescription, and dispensation of opioids for animals has been investigated. Piper et al. analyzed data from approximately 200 registrants nationwide for distribution of Schedule II and III opioids to animals from 2006 to 2019 in US veterinary teaching institutions [40]. By weight, codeine was the predominant dispensed opioid with a peak of approximately 2200 g in 2017, which then decreased to 1200 g in 2019. Hydrocodone followed codeine with a maximum of 550 g in 2010, which declined to 160 g in 2011. Oxycodone went from 233 g in 2006 to less than 5 g in 2012, and meperidine reached 55 g in 2006 to then dropped to 10 g in 2019. The distribution of morphine, hydromorphone, and oxymorphone was relatively stable during 2006–2019. However, the distribution of methadone increased from approximately 9 g in 2006 to 155 g in 2019. The distribution of fentanyl analogs peaked at 380 mg for remifentanil in 2008, 50 mg for sufentanil in 2009, and 40 mg for alfentanil in 2013. By percentage of total morphine milligram equivalents (MME), the top seven Schedule II and III opioids distributed in 2019 were methadone (42.3%), fentanyl (35.4%), codeine (6.4%), hydromorphone (5%), morphine (4.5%), hydrocodone (4%), and buprenorphine (2.4%). Despite the highly variable temporal pattern for individual opioids, a decline in hydrocodone use (which preceded its up‐scheduling from Schedule III to II), a recent decline in codeine, a decline in fentanyl use from 2013, and a gradual increase in methadone distribution were evident. The overall use of opioids by MME was relatively stable from 2014 to 2019, with only a 5.7% reduction. A limitation of this study was the lack of information on the Schedule IV opioids tramadol and butorphanol. Table 23.1 Potential vet‐shopping behaviora by US pet owners from 2014 to 2019. Source: Adapted from Chua et al. [37]. a Defined as the occurrence of dispensed controlled substance prescriptions from ≥ 4 veterinarians during the year. b The numbers in the second through fourth rows in this section do not add up to the number in the first row because (1) the second through fourth rows are not mutually exclusive (e.g., a patient could have opioid prescriptions from ≥ 4 veterinarians and benzodiazepine prescriptions from ≥ 4 veterinarians) and (2) some patients did not receive prescriptions for any given medication class from ≥ 4 veterinarians, but still received controlled substance prescriptions from ≥ 4 veterinarians (e.g., opioid prescriptions from two veterinarians and benzodiazepine prescriptions from two other veterinarians). Kogan et al. surveyed 697 veterinarians practicing in the US in 2018 and 97.8% used opioids in‐clinic, 80.3% dispensed them, and 70.3% prescribed them [35]. Buprenorphine, butorphanol, hydromorphone, methadone, morphine, injectable fentanyl, and tramadol were the most frequently administered opioids in‐clinic. Buprenorphine, tramadol, and hydrocodone were the most frequently dispensed opioids to clients, and tramadol, buprenorphine, and hydrocodone were the most frequently prescribed opioids to outside pharmacies. Overall, 99.7% of respondents used opioids in‐clinic, dispensed, or prescribed them. Buprenorphine (96.0%), tramadol (90.8%), butorphanol (90.5%), hydromorphone (71.7%), hydrocodone (61.4%), morphine (44.5%), fentanyl patch (27.3%), fentanyl injectable (21.4%), methadone (10.9%), and oxymorphone (5.6%) were the most frequently mentioned opioids. The greater use of μ‐opioid agonists in‐clinic compared to prescribing or dispensing of these drugs may be due to concerns about injectable opioids leaving the clinic, lack of efficacy of oral μ‐opioid receptor agonists in dogs and cats, and veterinarian concern over diversion of potent opioid drugs. During 2007–2017, veterinarians practicing at a small animal veterinary teaching hospital prescribed a total of 1,051,836 tramadol tablets, 97,547 hydrocodone tablets, 38,939 codeine tablets, and 3153 fentanyl patches to dogs (73.0%), cats (22.5%), and exotic species (4.5%). Although prescribing of tramadol tablets and fentanyl patches decreased, overall MME use increased by 41.2%, whereas visits only increased by 12.8% over the study period. The large and increasing volume of opioids prescribed at this veterinary teaching hospital highlights parallel concerns about excessive opioid prescribing in humans [41]. Increasing opioid prescribing trends were also found in veterinary practices using pharmacies to fill pet medications and reporting to West Virginia’s PDMP between January 2008 and June 2020. Veterinary practices reporting to West Virginia’s PDMP ranged from 1 in 2008 to 61 in 2019. Sixty‐nine veterinary practices submitted 7919 opioid prescriptions: 96.4% for tramadol, 1.4% for codeine, 1% for fentanyl, 1% for hydrocodone–acetaminophen, and 0.2% for morphine. Mandates in the PDMP and the introduction of tramadol as a controlled substance in 2014 may have contributed to the increasing trend in opioid prescribing [42]. Using IQVIA Longitudinal Prescription Datasets, which reports all prescriptions dispensed from 92% of US retail pharmacies and 70% of US mail‐order pharmacies, a mean of 1,110,594 (standard deviation 104,109) controlled substance prescriptions from veterinarians were dispensed annually from 2014 through 2019. During this period, tramadol accounted for the highest share of opioid analgesic prescriptions (92.6%), followed by codeine (2.9%), and hydrocodone (1.9%). Opioid analgesic dispensing peaked in 2016 (290,809 prescriptions) before declining in 2019 (184,364 prescriptions). Hydrocodone products accounted for 99.6% of opioid cough‐and‐cold medication prescriptions, while codeine products accounted for the other 0.4%. Opioid cough‐and‐cold medication dispensing doubled between 2014 and 2019, from 106,663 to 211,960 prescriptions [37]. Veterinarians require opioids for the practice of modern veterinary medicine. However, opioids carry risks to both animal patients and humans. These risks must be carefully managed at the individual and system levels, and it should be recognized that both patients and their owners can be harmed by clinical decisions to prescribe or not to prescribe opioids. There are times when opioids are the best medical choice and ensuring that veterinarians can provide these drugs to their patients is critically important. Paradoxically, in the midst of an opioid crisis, veterinarians have struggled to obtain opioids for their patients and have relied on alternative multimodal analgesic and anesthetic plans, sometimes without opioids. In 2018, 83% and 59% of 697 veterinarians practicing in the US reported difficulties obtaining opioids for use in‐clinic or to dispense to clients between May and October and in October alone, respectively. Conversely, 29% and 22% reported difficulties prescribing opioids to clients between May and October and in October alone, respectively. The most difficult opioids to obtain were hydromorphone, morphine, injectable fentanyl, oxymorphone, and methadone, all Schedule II drugs. The opioid shortage moderately or significantly affected the ability of 75% and 73% of veterinarians to provide optimal analgesia and anesthesia to their patients, respectively. Although only 11% of veterinarians canceled or postponed any procedures between May and October due to the opioid shortage, 45% discussed the opioid shortage with clients in situations where their pet required opioid treatment [35]. Although many believe that an opioid crisis could not happen outside the US, Canada has had a similar explosion of opioid prescribing and is facing an opioid epidemic. In both countries, more than 600,000 opioid overdose fatalities have been recorded since 1999 with a record peak in 2020: 70,168 deaths in the US and 6306 deaths Canada [32]. Furthermore, pharmaceutical companies based in the US are actively expanding opioid markets worldwide and are using tactics now banned domestically. This may partially explain the disproportionate distribution of controlled opioids with 21 countries consuming 89% of all controlled opioids between 2015 and 2017 and recent sharp increases in opioid prescribing in Australia, the Netherlands, Spain, Israel, Iceland, England, and Brazil [32,34,43]. A 2021 survey of veterinarians in the United Kingdom (UK) showed that 88.2% of respondents agreed with the statement “some drugs prescribed by veterinary professionals are at risk of misuse.” Of these, 29.9% had suspected a client of taking a drug prescribed to their animal, and 20.1% had suspected veterinary staff of taking a veterinary prescription medicine. The opioids tramadol, fentanyl (transdermal patch), buprenorphine, and codeine were in the top 10 drugs identified at risk of misuse by humans when prescribed by UK veterinarians [44]. Only 10.5% of respondents had received formal training regarding recognizing, preventing, or dealing with suspected drug misuse involving staff or clients, and 95.6% felt they would benefit from additional training or guidance [44]. Thus, veterinarians worldwide may be a source of prescription opioid misuse and training about this issue is required. The Nomenclature and Standards Committee of the International Union of Basic and Clinical Pharmacology approves the use of the well‐established Greek symbol terminology for the three types of opioid receptors: μ, δ, and κ. Also approved are the symbols spelled out (mu, delta, and kappa), and the acronyms MOP, DOP, and KOP where OP stands for opioid peptide [45]. The genes encoding these receptors are the opioid receptor mu 1 (OPRM1), opioid receptor delta 1 (OPRD1), and opioid receptor kappa 1 (OPRK1), respectively [45]. The principal endogenous agonists are: (1) β‐endorphin (derived from proopiomelanocortin) for the μ‐opioid receptor; (2) leucine‐ and methionine‐enkephalin (derived from proenkephalin) for the δ‐opioid receptor; and (3) big dynorphin and dynorphin A (derived from prodynorphin) for the κ‐opioid receptor [45]. The nociceptin/orphanin FQ (N/OFQ) or nociceptin opioid peptide (NOP) receptor is the fourth member of the opioid receptor family, and it is activated by the peptide of the same name (i.e., N/OFQ). Despite sharing high structural homology with conventional opioid receptors, N/OFQ is considered opioid‐related because it displays distinct pharmacology [45]. This receptor modulates various central processes including pain, learning and memory, emotional states, neuroendocrine control, food intake, and motor control [46]. Because no drugs interacting with this receptor are available for clinical use, it is beyond the scope of this chapter to discuss this receptor further. Opioid receptors belong to the family of G‐protein‐coupled receptors. Upon activation by an agonist, opioid receptors change their conformation causing the Gαi/o subunit to dissociate from the receptor and the Gβγ dimer. The guanosine triphosphate bound to the Gαi/o subunit is hydrolyzed to guanosine diphosphate rendering the Gαi/o subunit active, which, in turn, inhibits adenylate cyclase and cAMP‐related pathways. Voltage‐gated Ca2+ channels are inhibited through a Gαi/o‐GTP‐tyrosine kinase pathway in a voltage‐independent manner and by Gβγ dimers in a voltage‐dependent manner. The Gβγ dimer also activates G‐protein‐coupled inwardly rectifying K+ (GirK) channels [47]. This increases the membrane potential rendering the cell less susceptible to depolarization. If opioid receptor activation continues long term, Gβγ dimers recruit G‐protein receptor kinase (GRK) to the receptor for phosphorylation. This changes receptor conformation allowing β‐arrestin to dock and subsequently recruit the endocytosis machinery causing receptor internalization. Receptors are either recycled after some time to the cell membrane or transported to proteasomes and lysosomes for degradation. The latter causes receptor downregulation, which decreases the number of opioid receptors available for activation in the cell membrane. Thus, opioid receptors undergo homologous desensitization becoming less responsive to continuous or repeated sustained activation by opioid agonists, which may contribute to the development of tolerance. Interestingly, opioid receptor activation has been demonstrated to occur not only at the cell membrane, but within cytoplasmic compartments [48]. Receptor regulation and desensitization is dependent on cellular location, cell type, and agonist type. The fact that different agonists produce different pharmacologic responses when acting at the same opioid receptor led to speculation that multiple subtypes of μ‐, δ‐, and κ‐opioid receptors existed [49,50]. However, molecular cloning has failed to confirm the existence of such subtypes, and knockout of each of these receptors results in a loss of ligand binding and function associated with that receptor [50]. Instead, differences in response are mediated by rather more complex opioid receptor functions. These include spatiotemporal specificity in opioid receptor cellular signaling, ligand‐directed biased signaling, positive and negative allosteric modulation of ligand binding and signaling, homo‐ and heterodimerization of different opioid receptors, and the existence of splice variants with different ligand specificity [51,52]. Alternative splicing of opioid receptor genes may contribute to interindividual variation in response to opioid treatment. Various single‐nucleotide polymorphisms (SNPs) of the OPRM1 gene have been identified in dogs [53,54]. Interestingly, the C‐15A SNP, which had high prevalence in the Alaskan Malamute, Siberian Husky, and Labrador Retriever dogs that were studied, seems to play a role in opioid‐induced dysphoria [53]. Opioid receptors are distributed throughout the body, including the brain, spinal cord, peripheral sensory nerve terminals, enteric neurons, genitourinary tract, synovial membranes, leukocytes, and blood vessels. Therefore, the activation of opioid receptors induces various effects (Table 23.2). The distribution of opioid receptors in key areas of pain transmission and modulation contributes to the analgesic effects of opioid agonists. However, μ‐ and κ‐opioid receptor distribution in the brain differs by species [55], and μ‐opioid receptor density in the spinal cord increases in chronic pain states [56]. Activation of opioid receptors in extravascular leukocytes by exogenous opioids triggers secretion of endogenous opioid peptides from the immune cells contributing to the analgesic effect of exogenously administered opioid receptor agonists [57]. Table 23.2 Physiological effects associated with stimulation of opioid receptor types. Opioids can also be classified according to the receptor or receptors they interact with, as well as the effect elicited (e.g., concentration–response or dose–response relationship). Classically, a full agonist produces a dose‐dependent increase in effect until maximum stimulation of the receptor is achieved (Fig. 23.1A). A partial agonist also produces a dose‐dependent increase in effect, but plateaus at a maximal response that is less than that achieved with a full agonist (Fig. 23.1B). Figure 23.1 A. Dose–response curves for two opioids with equal efficacy but different potencies. This example could represent the full agonists morphine (line B) and fentanyl (line A). The dose of fentanyl needed to achieve a given effect is lower, but the maximum effect of both fentanyl and morphine will be similar. B. Dose–response curves for two opioids with different efficacies and potencies. This example could represent the full agonist morphine (solid line) and partial agonist buprenorphine (dashed line). Despite buprenorphine being a more potent drug with high affinity for opioid receptors, the maximum effect attained with morphine is greater. An antagonist binds to a receptor with high affinity but produces no intrinsic effect and inhibits the binding of agonists (both endogenous and exogenous). Administration of an antagonist will, therefore, “reverse” the receptor‐mediated effects of an agonist by inhibiting agonist binding and displacing previously bound agonist due to its greater affinity for the receptor. Most opioid receptor antagonists are considered competitive antagonists based on their lack of intrinsic activity and the fact that high concentrations of an agonist can overcome antagonism and still produce a maximum effect (i.e., the antagonist shifts the agonist’s dose–response curve to the right). Administration of a partial agonist can act as an antagonist by partially reversing the effects of a full agonist (also shifting the dose–response curve to the right) and this approach may be preferred over an antagonist in certain situations (e.g., if maintenance of some analgesia is desired) [58]. The potency of an agonist describes the relative dose needed to elicit a response. For opioids, the response of interest is usually analgesia measured as a change in withdrawal latency or increased threshold to a noxious stimulus. For example, fentanyl is a more potent opioid than morphine, which means the dose of fentanyl (0.01 mg/kg) needed to produce an equivalent analgesic response to morphine (1 mg/kg) is lower (Fig. 23.1A). Note that potency does not describe duration of effect or efficacy. In the example of fentanyl (0.01 mg/kg) and morphine (1 mg/kg), both opioids produce equivalent maximum analgesic effects, but duration of analgesia is considerably shorter with fentanyl. While fentanyl and morphine can be equally efficacious analgesics since both are μ‐opioid receptor agonists, the typical dose of morphine is 100‐fold greater than that of fentanyl since it is less potent. Pharmacokinetics can be defined simplistically as “what the body does to a drug,” and it can be divided into absorption, distribution, metabolism, and excretion. These processes depend on the drug itself and its formulation, as well as the species, age, disease status, and other factors specific to the individual. By determining drug concentrations, usually in blood plasma, pharmacokinetic studies can estimate the rate and extent of drug absorption, the persistence of drug after a given dose, and sometimes the potential for drug interactions. Table 23.3 summarizes selected pharmacokinetic parameter estimates for commonly used opioids in domestic species. Absorption is the movement of a drug from its site of administration into the systemic circulation. Once in systemic circulation, the drug is considered to be bioavailable. By definition, a drug that is administered intravenously (IV) bypasses absorption (i.e., it is already in the systemic circulation). Opioids are very lipophilic, which allows them to move easily across cell membranes. Generally, they are rapidly and extensively absorbed after intramuscular (IM) or subcutaneous (SC) administration. Differences in onset of action and duration of effect have been reported with SC administration of hydromorphone and the “traditional” 0.3 mg/mL formulation of buprenorphine in cats, with the SC route associated with shorter duration antinociception and analgesia compared to IM or IV routes [98,99]. However, certain controlled‐release opioid formulations (e.g., sustained‐release buprenorphine for SC administration) produce prolonged effects compared to readily absorbable formulations (e.g., “traditional” 0.3 mg/mL buprenorphine) [20]. After oral (PO) or rectal administration, opioids can move across membranes in the gastrointestinal tract and enter local capillaries and then travel to the portal vein and liver where they usually undergo extensive metabolism [100]. This is known as “first‐pass metabolism” and renders the drug ineffective when administered at standard doses. The oral transmucosal (OTM) route bypasses first‐pass metabolism, and some buprenorphine formulations have demonstrated high bioavailability with this route of administration [30,101]. Table 23.3 Selected pharmacokinetic parameters of some clinically relevant opioids in awake, healthy animalsa. a t½, elimination half‐life; Vdss, volume of distribution at steady state; Cl, clearance; F, bioavailability; IV, intravenous; IM, intramuscular; SC, subcutaneous; CRI, constant rate infusion; PO, oral; OTM, oral transmucosal. a Values are mean ± standard error or median and (range). b High‐concentration formulation (1.8 mg/mL). c Values are median and (25th–75th percentiles). Transdermal administration of specially formulated opioids can result in absorption across the stratum corneum. Transdermal fentanyl patches designed for humans have been used with some success in veterinary animal species [102]. A buprenorphine transdermal matrix patch has also been evaluated in cats and, while adequate plasma concentrations were obtained, no effect on thermal threshold was measured [103]. Limitations associated with transdermal patch delivery of opioids to animals such as adherence to animal skin, accurate dosing, and potential for misuse deter their use. A novel transdermal buprenorphine formulation approved by the US Food and Drug Administration (FDA) results in plasma drug concentrations that persist for 7 days after a single administration in cats [104]. Regional or local opioid administration has been used to maximize therapeutic efficacy and minimize systemic adverse events. For instance, epidurally administered opioids are used to induce analgesia with minimal excitation in horses. Interestingly, morphine concentrations were higher in blood plasma than in the cerebrospinal fluid for 24 h after epidural administration and hysteresis, a delay in analgesia compared to peak drug concentration, of about 4 h occurred [105,106]. In a similar way, buprenorphine was detectable in the synovial fluid of inflamed radiocarpal joints of horses at high concentrations for up to 24 h after intra‐articular administration, but only for up to 6 h and at low concentrations after IV administration; both routes of administration produced similar blood plasma drug concentrations [107]. For a drug to induce its therapeutic effects, it needs to reach its site of action at effective drug concentrations. The central nervous system (CNS) is where opioids induce their analgesic, antitussive, and sedative effects. Although most opioids penetrate the CNS, not all induce CNS effects. The μ‐opioid receptor agonist loperamide is actively excluded from the CNS and is sent back into the circulation by P‐glycoprotein efflux pumps. Consequently, loperamide generally acts peripherally and is used as an antidiarrheal. However, dogs with non‐functional P‐glycoprotein due to homozygous mutation of the ABCB1‐1∆ gene display CNS effects after loperamide administration [108]. Various opioids are substrates for P‐glycoprotein and patients with homozygous or heterozygous ABCB1‐1Δ gene mutation may have longer lasting and more intense effects than wild‐type gene carriers [109]. Interestingly, morphine accumulated more in the brain than in blood plasma of near‐term pregnant sheep (1.19 ratio) and premature lambs (1.89 ratio) suggesting lack of an efflux transporter across the blood–brain barrier in sheep [90]. In addition to the blood–brain barrier, opioids can be transported across other specialized barriers such as the placenta and subsequently enter the fetal circulation [90,91,110]. Opioids have large volumes of distribution, but there are differences between drugs (see Table 23.3). These differences are due to the biochemical characteristics of the drug and the relative organ blood supply. For instance, fentanyl (4.28 log P) is more than 1000‐fold more lipophilic than morphine (1.07 log P) and distributes faster into and out of highly perfused tissues such as the CNS [111]. Therefore, after IV administration, fentanyl has a faster onset, but shorter duration of analgesia compared to morphine [112]. These differences in lipophilicity can be exploited when selecting an opioid for epidural administration, with less lipophilic opioids producing longer lasting analgesia compared to more lipophilic opioids when administered by this route [105,113]. When opioids are administered into or close to their intended site of action, such as with epidural or intra‐articular administration, their therapeutic effects are terminated by absorption of the opioid into the local vasculature before they are metabolized and eliminated. Opioids are extensively metabolized by the liver. Phase I reactions, which include oxidation (via the cytochrome P450 system), reduction, and hydrolysis, convert the parent drug to a more polar‐ or water‐soluble molecule. Phase II reactions increase the water solubility of a molecule by conjugation of subgroups usually with glucuronate, acetate, or sulfate. Glucuronidation is the main metabolic pathway for opioids [78–80,114]. However, cats are deficient in this pathway and use conjugation with sulfate to metabolize morphine [115]. In contrast to humans, opioids have low oral and rectal bioavailability in animals due to extensive first‐pass metabolism, usually rendering them ineffective when administered by these routes at standard doses [64,116]. Opioid metabolizing enzymes are also found in other tissues such as the intestine and the brain [117]. Differences in individual responses to opioids may be mediated by genetic polymorphisms in metabolizing enzymes [109]. In general, drug metabolites are less active than the parent drug; however, many opioid metabolites maintain some pharmacologic activity. In many species, for instance, morphine is conjugated with glucuronate to form morphine‐3‐glucuronide and morphine‐6‐glucuronide. Although morphine‐6‐glucuronide is regarded as the active metabolite, morphine‐3‐glucuronide may be active too, and interactions between these metabolites and the parent drug may contribute to the effects of morphine [81]. Tramadol is metabolized to its active O‐desmethyltramadol metabolite [116]. Some opioids are pro‐drugs and become active drugs after being metabolized. For example, hydrocodone is metabolized to the active compound hydromorphone and the less active norhydrocodone [79,80]. Some drugs induce or inhibit drug metabolizing enzymes and interactions between these drugs and opioids may have important clinical implications. After oral tramadol administration in dogs, for instance, the enzyme inhibitor fluconazole increased by more than 30‐fold both tramadol and O‐desmethyltramadol blood plasma and urine concentrations [118]. Similarly, the enzyme inhibitors ketoconazole and cimetidine increased mean oral tramadol bioavailability from 2.6% to 18.2% and 20.3% and mean maximum blood plasma concentration from 22.9 ng/mL to 109.9 ng/mL and 143.2 ng/mL, respectively [119]. On the other hand, in dogs, the enzyme inducer grapefruit juice decreased the maximum blood plasma tramadol concentration (Cmax) (490 versus 270 ng/mL), delayed the time that Cmax occurred (Tmax) (1.33 versus 1.7 h), and decreased the area under the curve (AUC) (11,610 versus 5890 h·ng/mL) [120]. Also in this last study, O‐desmethyltramadol Cmax was increased (80 versus 130 ng/mL), Tmax occurred faster (1.36 versus 0.86 h), and AUC was decreased (340 versus 160 h·ng/mL) [120]. In general, very little of the opioid dose is excreted as the parent drug via the urine or feces. Opioid metabolites are often eliminated in the urine, but some can be eliminated in the feces by biliary secretion. Patients with hepatic or renal impairment may have altered opioid clearance and may require dose adjustments to avoid drug accumulation and the potential for adverse events [121]. Opioid agonists activate reward processes in the absence of significant pain and motivate repeated use of escalating doses of the drugs simply for pleasure. This alters the brain so that it functions more or less normally when the drugs are present and abnormally when they are not. Two clinically important results of this alteration are opioid tolerance and dependence. Opioid tolerance is the need to take higher dosages of drugs to achieve the same therapeutic effect. Opioid dependence is the need to use an opioid agonist to avoid a withdrawal syndrome. Opioid withdrawal symptoms, together with social and genetic effects, contribute to the development of opioid addiction. Opioid addicts require detoxification and pharmacological treatment, usually with methadone, levo‐α‐acetylmethadol, buprenorphine, or naltrexone, which must be accompanied by psychosocial therapy [122]. The exact mechanisms involved in the development of opioid tolerance and reward processes remain to be elucidated. However, opioid tolerance develops in the midbrain periaqueductal gray (PAG) and rostral ventral medulla descending system. Increased activation of adenylate cyclase in γ‐aminobutyric acid (GABA) interneurons in the PAG results in protein kinase A‐mediated phosphorylation of Ca2+ channels and, therefore, increased calcium conductance. The overall effect is increased GABA release and, therefore, stronger inhibition of PAG output neurons [123]. Opioid reward takes place in the mesolimbic dopamine system where opioid agonists generate signals in the ventral tegmental area that result in the release of dopamine in the nucleus accumbens inducing feelings of pleasure. Other areas of the brain create memories that associate these good feelings with the circumstances and environment in which they occur. These conditioned associations lead to the craving for drugs when the abuser reencounters those people, places, or things, and they drive abusers to seek out more drugs [122]. Opioid tolerance usually precedes opioid dependence, and it may be overcome by increasing the dose or administering a different opioid agonist (i.e., opioid rotation). However, increasing the dose increases the risk of opioid‐induced adverse events. Opioid tolerance has been extensively studied in experimental pain‐free dogs and cats (Table 23.4). Daily administration of 3 mg/kg of morphine to dogs initially induced sedation lasting up to 24 h, which gradually decreased in both degree and duration. Sedation was increased by increasing the dose, but within three weeks of starting treatment, morphine did not induce sedation despite the increased doses. Complete tolerance to morphine’s emetic effects developed within 10 days [124]. Although the route of administration was not stated in this report, the dosage used was higher than the recommended morphine dosage for clinical use in dogs. SC administration of morphine at lower dose rates (twice a day for 7 days at 0.5 and 2.5 mg/kg or infused at 1–5 mg/kg/day for 8 days) induced faster tolerance development in dogs [125]. In cats, however, administration of high doses of morphine (1–4 mg/kg/day IV) for 7 days induced minimal tolerance [126]. Procedural details rather than species differences between dogs and cats may explain the differences in the development of tolerance. The cat study also suggests that tolerance can be prevented by large opioid doses. Cats exposed to rounds of increasing lower doses of morphine (1–2 mg/kg/day SC divided into morning and evening doses over 24 to 30 days) developed tolerance, which was overcome by increasing the morphine dose [127]. Opioid dependence, determined by appearance of withdrawal signs, was also reported in the abovementioned studies. One to two months after morphine (3 mg/kg/day) administration, dogs became irritable and fought frequently at the end of the 24‐h administration, which was overcome by shortening the dosing interval or increasing the dose [124]. After ceasing morphine administration, 8 out of 10 dogs showed mild signs of opioid withdrawal characterized by rigidity with spastic gait, muscle twitching, retracted belly, sleepiness, salivation, diarrhea, and vomiting; one dog showed moderate signs which also included restlessness, piloerection, gnawing at objects, whining, hiccough, panting, and growling; and one dog showed marked signs which additionally included jerky respiration, and being irritable, belligerent, and very noisy. Maximal signs were seen three days after morphine withdrawal and all signs disappeared after 8–10 days. When morphine withdrawal was induced by nalorphine administration, seven, nine, and six dogs showed slight, moderate, and marked withdrawal signs, respectively. 1 h after nalorphine administration, excitement was observed, and 1 h later, it had disappeared [124]. Similarly, after one week of morphine administration and withdrawal precipitated by subcutaneous naloxone (1 mg/kg) administration, dogs showed signs of hyperactivity, biting, licking, grooming, digging, tremors, nausea, hyperthermia, mydriasis, and increased wakefulness [125]. In cats, whole body shakes, head shakes, urination (often in a standing position), miosis, and catatonic‐like posturing resulted from naloxone (0.5 mg/kg IV)‐precipitated withdrawal in cats maintained for 12 days on IV morphine (1–4 mg/kg/day) [126]. After being exposed to 24‐ to 30‐day‐rounds of increasing doses of morphine, cats slept most of the day and ate very little during a 2‐day withdrawal period. This behavior was similar during a 2‐week withdrawal period, with appetite and activity levels improving during the second week. Fatigue, muscle weakness, and pupillary constriction were noted during the first and second day of withdrawal periods. Mild muscle tremors were observed, especially early in the final withdrawal period [127]. Interestingly, plasma cortisol levels increased in these cats at the beginning of the withdrawal periods and decreased to baseline levels when morphine was administered again or 6 weeks after morphine withdrawal [127]. In another cat study, psychological dependence to opioids was demonstrated by self‐administration of morphine [128]. Together, these studies suggest that opioid‐dependent cats will self‐medicate with opioids, if available, and that opioid withdrawal is stressful. Table 23.4 Effects of morphine on opioid‐induced tolerance in dogs and cats. IV, intravenous; SC, subcutaneous. Repeated exposure to opioid agonists may cause an exacerbated pain state known as “opioid‐induced hyperalgesia” (OIH). This phenomenon was described as early as 1870 [129]; however, the first prospective, controlled clinical trial demonstrating OIH was published in 2006 [130]. In this trial, participants suffering from lower back pain developed hyperalgesia, as assessed by a cold pressor test, after one month of oral morphine therapy [130]. The exact mechanisms by which OIH occurs are not fully understood but involve maladaptive plastic changes in opioid‐responsive pain processing pathways. Some of these changes include: (1) activation of descending pain facilitation from the rostral ventromedial medulla by increased activity of cholecystokinin and “on‐cell” firing which in turn activates spinal pathways to upregulate dynorphin and enhance spinal nociceptive inputs; (2) spinal glutaminergic systems, via sustained activation of N‐methyl‐D‐aspartate receptors, which sensitize dorsal horn neurons to intensify pronociceptive ascending pathways; and (3) increased activity of catechol‐O‐methyltransferase with subsequent higher breakdown of dopamine and noradrenaline enhancing nociceptive responses [123,131]. Neuroimmune cell communication has also been implicated in OIH. Upregulation of P2X4‐purinergic receptors in microglia and subsequent release of brain‐derived neurotrophic factor activated tropomyosin receptor kinase B on spinal dorsal horn neurons, which downregulated potassium‐chloride co‐transporter KCC2, shifting GABA receptor activation from inhibitory to excitatory [132]. Few studies have investigated OIH in domestic animal species. Two studies demonstrated a κ‐opioid‐receptor‐mediated pontine‐medullary hyperalgesic system in dogs [133,134], and one study reported thermal hyperalgesia after morphine administration in cockerels [135]. Although naloxone reversed the development of hyperalgesia in these three studies, hyperalgesia was induced after a single systemic or local administration of an opioid agonist. Opioid tolerance, dependence, withdrawal, or OIH has not yet been systematically assessed in veterinary clinical cases. However, these phenomena may not present practical concerns as opioids are mainly administered for acute uses and long‐term use is not recommended for chronic pain control [136,137]. Furthermore, approved opioid medications in animals maintain clinical efficacy for up to 4 days after a single administration [104]. Opioid analgesics have been the cornerstone for perioperative pain management in veterinary medicine for several decades. A comprehensive understanding related to their molecular and cellular mechanism of action allows the clinician to maximize the efficacy of opioid analgesics and mitigate their unwanted adverse effects. Opioids, acting as endogenous (e.g., endorphins, enkephalins, dynorphins, endomorphins, and nociceptins) or exogenous (e.g., methadone, hydromorphone, morphine, etc.) ligands, bind to μ‐, δ‐, and κ‐opioid receptors as well as nociceptin/orphanin FQ receptors producing a desired effect [138]. Opioid receptors are structurally classified as seven transmembrane G‐protein‐coupled receptors and are located at supraspinal (e.g., cortex, thalamus, hypothalamus, locus coeruleus, periaqueductal gray, amygdala, and cingulate gyrus), spinal (e.g., dorsal horn of the spinal cord), and peripheral tissue (e.g., dorsal root ganglia, peripheral nerve endings of sensory neurons, inflamed tissue, and immune cells) levels [139,140]. Binding of an exogenous or endogenous opioid ligand to opioid receptors results in a conformational change that allows intracellular coupling of Gi/Go proteins to the C terminus of the opioid receptor (Fig. 23.2) [140]. This activation dissociates the trimeric receptor into several active subunits (Gα, Gβ, Gγ). The Gα subunit then binds to adenylate cyclase preventing the formation of cyclic adenosine monophosphate, an important secondary messenger associated with autonomic tone [138]. In addition, activation of Gα subunits open inwardly rectifying potassium channels (Kir3) resulting in an efflux of potassium ions. This effect hyperpolarizes the cell membrane, preventing neuronal excitation and/or the duration of the action potential. Gβ and Gγ subunits directly interact with voltage‐gated calcium channels (VGCC) decreasing calcium influx via closure of pre‐ and postsynaptic calcium channels, thereby decreasing intracellular calcium concentrations. Within laminae I and II of the dorsal horn of the spinal cord, a reduction of calcium influx reduces the spinal release of glutamate and substance P [141]. Similar to the effect observed with Gα on Kir3 channels, the action potential duration and the release of pronociceptive neuropeptides is reduced [138,140]. In the PAG, binding of exogenous opioids or endogenous opioid peptides to opioid receptors inhibits GABAergic interneurons, activating descending inhibitory pathways that project to the dorsal horn of the spinal cord, thereby modulating transmission of the nociceptive impulse [142]. Stimulation of opioid receptors on peripheral terminals of primary afferent nerves associated with inflamed tissue can also induce potent antinociceptive effects [143–145]. Endogenous and exogenous opioids bind to opioid receptors synthesized in the dorsal root ganglia that are transported along intra‐axonal microtubules to peripheral sensory neuron terminals. This results in inhibition of peripheral ion channels (e.g., transient receptor potential vanilloid‐1 [TRPV1], hyperpolarization‐activated cyclic nucleotide‐gated [HCN], sodium, acid‐sensing ion [ASIC] channels) and substance P [140]. Increased neuronal electrical activity and production of inflammatory cytokines associated with inflamed peripheral tissue stimulate mRNA and specific proteins to upregulate production of opioid receptors and induce differential regulation of opioid receptor types [140]. This has been observed more consistently with μ‐opioid receptors [146]. Similar influences on opioid receptor expression are observed at sites of nerve injury and in tissues innerved by damaged nerves [147]. Figure 23.2 Opioid receptor signaling and recycling. A. Opioid receptor ligands induce a conformational change at the receptor that allows coupling of G‐proteins to the receptor. The heterotrimeric G‐protein dissociates into active Gα and Gβγ subunits, which can inhibit adenylate cyclase and reduce cAMP, decrease the conductance of voltage‐gated Ca2+ channels, or open rectifying K+ channels. In addition, the phospholipase C (PLC)/phosphokinase C (PKC) pathways can be activated to modulate Ca2+ channel activity in the plasma membrane. B. Opioid receptor desensitization and trafficking is activated by G‐protein‐coupled receptor kinase (GRK). After arrestin binding, the receptor is in a desensitized state at the plasma membrane. Arrestin‐bound receptors can then be internalized via a clathrin‐dependent pathway, and either be recycled to the cell surface or degraded in lysosomes. Source: Stein [140], Annual Reviews. Opioid analgesics administered locally to inflamed tissues bind to peripheral opioid receptors and dampen excitatory impulses of sensory nerves similar to that observed at presynaptic opioid receptors in the spinal cord. Thus, peripheral analgesic effects are only produced when opioid agonists are administered to tissues that are inflamed or affected by nerve injury. Multiple effective routes of administration contribute to the versatility of opioids as analgesics in a variety of clinical settings. This is largely a function of the diverse locations of opioid receptors within the neuroaxis, which allows for effective pain control when opioids are administered peripherally (intra‐articular), neuraxially (intrathecal and epidural), systemically (IV, IM, SC, and OTM), or transdermally. Choosing the appropriate opioid for pain management depends on several patient‐related and drug‐specific considerations (Box 23.1). Opioids can be administered in combination with other perioperative agents to produce neuroleptanalgesia (e.g., a benzodiazepine or acepromazine with an opioid), and as part of multimodal analgesic protocols. Full μ‐opioid receptor agonists are often preferred over partial or mixed agonists due to their efficacy in managing acute surgical pain. A higher fraction of opioid receptor occupancy is often required with partial agonists (i.e., buprenorphine) to achieve an analgesic response of equivalent magnitude when compared to full agonists. In most domestic species, full μ‐opioid receptor agonists provide more profound analgesic effects when compared to partial agonists [148–150], while both full and partial agonists provide superior analgesia when compared to mixed agonist–antagonist agents [151–155]. This may not always be the case with certain species of birds; however, this remains controversial. Despite differences among full agonists, partial agonists, and mixed agonist–antagonists, all three mediate analgesia to some degree and partial or mixed agonists can provide effective therapy in patients with moderate or mild pain, respectively. It has been generally accepted that administration of a partial μ‐opioid receptor agonist (e.g., buprenorphine) produces an analgesic ceiling effect [156]. However, the classic “bell‐shaped” dose–response curve for buprenorphine reported in some early rodent studies has not been reproduced in more recent animal studies or in humans. Some evidence suggests this ceiling effect may be more relevant to non‐analgesic effects, such as respiratory depression, rather than analgesia [157]. Similar findings may apply to other opioids such as butorphanol [158–160]. Opioids induce dose‐dependent sedation in most species [161]. When combined with acepromazine, the rank order of opioids based on sedative intensity in dogs was methadone (0.5 mg/kg) > morphine (0.5 mg/kg) > butorphanol (0.15 mg/kg) > nalbuphine (0.5 mg/kg) [162,163]. Butorphanol (0.2 mg/kg), when combined and administered IV with dexmedetomidine (2 μg/kg), had a faster onset and greater magnitude of sedation when compared to methadone (0.2 mg/kg) in dogs [163]. Sedation associated with opioid administration does not always correlate with analgesia; thus, the clinician must assess the patient for appropriate pain control. For example, in two human trials, morphine‐induced sedation was a poor indicator of appropriate analgesia during IV titration [164] and did not ensure adequate pain relief postoperatively [165]. Opioid‐based procedural sedation protocols (e.g., an opioid combined with an α2‐adrenergic receptor agonist [166]) used for blood donation or diagnostic imaging are generally safe with minimal negative effects on diagnostic findings in healthy dogs [167,168] and cats [169–172]. The mechanism of opioid‐induced sedation is complex and not completely understood [173]. One theory is that opioids decrease sensory input and promote the probability of sleep [174]. Contrary to this theory, there is evidence that opioid administration disrupts the normal sleep–wake patterns by decreasing the amount of rapid eye movement (REM) sleep in animals [175,176]. Selectivity for the opioid receptor may also vary the influence on REM sleep. For example, intracerebroventricular administration of DAMGO, a selective μ‐opioid receptor agonist, inhibited REM sleep, whereas DPDE and U50,488H (μ/δ‐ and κ‐opioid receptor agonists, respectively) have minimal to no effect on REM cycles [176]. Spectral analysis of EEG recordings has been used to further understand the sedative effects of opioid receptor agonists when administered peripherally and centrally in animals. High‐amplitude, low‐frequency EEG recordings were observed in combination with a wide range of behaviors (e.g., stupor, immobility, catatonia, ataxia, increased locomotion, and hyperreactivity) [173]. Similar findings were observed in humans administered short‐acting μ‐opioid receptor agonists [173]. Evoked potentials have also been used to evaluate the effects of opioid receptor agonists in animals. In rats, small increases in amplitude and AUC of the cortical evoked potential were associated with morphine‐induced sedation [177]. Morphine administered to human cancer patients resulted in a delayed response to an auditory stimulus when compared to those that did not receive opioids [178]. In contrast, the administration of morphine to relieve chronic non‐malignant pain in humans resulted in enlarged evoked potentials with reduced reaction times [179,180]. Researchers from the latter study postulate that cognitive status improved because of the removal of pain as a mental stressor/distractor [179,180]. In addition to individual patient‐related factors, species also impacts the reliability of opioids to produce sedation. Cats have been reported to exhibit euphoric and/or dysphoric behaviors following opioid administration. Euphoria, defined as rolling, kneading with forepaws, vocalizing, and purring, is evident following hydromorphone, butorphanol, methadone, and buprenorphine administration in cats [58,181]. Butorphanol has also been reported to cause dysphoria (i.e., vocalizing, pacing, and staring) in cats [182,183]. Both situations hinder the ability to appropriately restrain patients for intravenous catheterization and routine diagnostic procedures. Cats also exhibit unique behavioral effects with the administration of morphine. “Morphine mania” or “feline mania” is a dose‐dependent (> 5–10 mg/kg) behavioral response characterized by behavioral excitation, motor agitation, and aggression [184,185]. Morphine administered to cats at 3 mg/kg intraperitoneally (still considerably higher than doses used clinically) resulted in three distinct behavioral stages: (1) the autonomic stage (0–15 min post‐drug administration) characterized by vocalization, salivation, licking, swallowing, and vomiting; (2) the quiet stage (15–60 min post‐drug administration) characterized by sitting, staring, mydriasis, and pricked pinnae; and (3) the head movement stage (30–60 min and decreasing within 5 h post‐drug administration) characterized by sitting, arousal, discrete head movements that appear in response to visual‐tracking, pouncing/avoidance paw movements, rocking, pivoting, and backing [186]. Minimal evidence of sleep, grooming, micturition, and defecation were noted during these stages [186]. Healthy dogs anesthetized with inhalant anesthetics and administered a μ‐opioid receptor agonist (i.e., fentanyl or morphine) may also exhibit signs of dysphoria during the recovery period [187,188]. The administration of a 0.5 μg/kg dexmedetomidine bolus IV over 10 min immediately prior to extubation in dogs administered fentanyl infusions and inhalant anesthetics can reduce the incidence of opioid‐induced dysphoria [189]. Horses also are reported to have excitatory responses following μ‐opioid receptor agonist administration (Table 23.2) [190]. Dose‐dependent increases in arousal and locomotor activity (i.e., foot stepping, spontaneous flexion of forelimbs, postural changes) are observed following 2.5, 5, and 10 μg/kg IV doses of fentanyl [191]. Excitation has also been observed following morphine, hydromorphone, pentazocine, meperidine, buprenorphine, and methadone administration in this species [13,192]. It has been suggested that opioid‐induced locomotor enhancement in horses is due to opioid and dopamine receptor stimulation [191], as the locomotor effects of fentanyl and morphine can be antagonized by naloxone (0.015–0.02 mg/kg IV) and acepromazine (0.10–0.16 mg/kg IV) [193]. Conclusions pertaining to opioid‐induced locomotion in horses include: (1) opioids induce eating behavior at low doses; (2) dose‐dependent locomotor activity with incoordination is observed at high doses; (3) the median effective dose for morphine to result in increased locomotion is 0.91 mg/kg, which is significantly higher than doses used to produce analgesia; and (4) there is variability in behavioral responses in the horse population [194]. It is difficult to determine if activation of different opioid receptor types plays a role in opioid‐induced excitation in horses. Butorphanol is reported to enhance locomotor activity associated with fentanyl administration [190]. However, yawning, ataxia, and staggering are more commonly observed following κ‐opioid receptor agonist administration [195]. In the author’s experience, butorphanol also results in head twitching following administration. In general, μ‐opioid receptor agonists promote increased locomotion when compared to κ‐opioid receptor agonists. Opioids increase parasympathetic tone and inhibit sympathetic tone leading to vagally mediated bradycardia and occasionally hypotension. Several predisposing risk factors may increase the likelihood of opioid‐induced bradycardia. These include the administration of β‐adrenergic blocking agents, calcium channel antagonists, α2‐adrenergic receptor agonists [196], inhalant anesthetics [197], muscle relaxants that lack parasympatholytic properties (e.g., vecuronium), high vagal tone (e.g., brachycephalic breeds) or various vagotonic physical stimuli (e.g., traction of ophthalmic muscles [198] or carotid massage [199]), high doses of opioids, and rapid IV bolus administration. Methadone’s chemical structure resembles that of calcium blocking agents and results in functional L‐type calcium channel blockade [200]. This is independent of its μ‐opioid receptor effects [200] and, therefore, may potentiate bradycardia [201]. Meperidine exhibits a slightly different action than other opioids (e.g., increased heart rate) due to its anticholinergic effects [202]. Conversely, opioids have been reported to increase heart rate in horses at clinically relevant doses [79]. Opioids generally exhibit a dose‐dependent effect on cardiac index (CI) that is associated with a reduction in heart rate (HR); however, this may be affected by species and the specific opioid administered. Morphine administered at 1 mg/kg IV to dogs resulted in a 26% and 19% reduction in HR and CI, respectively, at 120 min post‐administration. Methadone resulted in more pronounced depressant effects on HR and CI in conscious dogs compared to morphine at similar doses (i.e., 0.5 mg/kg methadone reduced HR by 19–28% and CI by 17–27%; 1 mg/kg methadone reduced HR by 32–46% and CI by 24–52%) [203]. Alterations in systemic vascular resistance index (SVRI) vary among opioids. In general, administration of opioids (e.g., methadone and buprenorphine) has been reported to increase SVRI via increased vasopressin concentrations in anesthetized and conscious dogs [197,203]. Morphine, however, has been reported to decrease SVRI [203], and butorphanol has been reported to have limited effects [204]. Similarly, meperidine has been reported to reduce SVRI due to its direct smooth muscle relaxant effect and indirect histamine releasing effect [205]. Opioids have been reported to alter the electrocardiogram and these effects may be exacerbated during inhalant anesthesia [206]. For example, methadone and hydromorphone prolong the PR and QT intervals when administered at clinically relevant doses in dogs. Opioids disrupt the delayed rectifier potassium current resulting in reduction of potassium efflux. This effect prolongs the action potential and delays ventricular repolarization, thus prolonging the QT interval on the electrocardiogram [207]. Delays in the PR interval following opioid administration have been known to result in bradyarrhythmias such as second‐degree atrioventricular (AV) block. The calcium blocking effects of methadone contribute to delayed depolarization of both the sinoatrial (SA) and AV nodal pacemaker cells [206]. Administration of an anticholinergic drug (e.g., glycopyrrolate or atropine) can be used to treat or prevent opioid‐induced bradyarrhythmias [208]. Opioids have been reported to exert their respiratory depressant effects at several supraspinal locations (Box 23.2) [209,210]. Opioid‐induced ventilatory depression is a result of interactions with supraspinal μ‐opioid receptors in the forebrain, superficial and deep brainstem tissue, and/or in the suprapontine tissue [209]. A large component of excitatory drive to the medullary rhythm generator (chemodrive) is routed through an area of the brain called the Parabrachial Nucleus/Kölliker‐Fuse Complex and assists in determining respiratory rate, while tidal volume is dependent on direct output projections from the retrotrapezoid nucleus to the preBötzinger Complex neurons [210]. Opioids depress Parabrachial Nucleus/Kölliker‐Fuse Complex activity in addition to acting at the nucleus of the solitary tract and the medullary raphe (Fig. 23.3) [210]. Clinically, opioids exert a dose‐dependent respiratory depressant effect resulting in respiratory acidosis and increased arterial carbon dioxide tension (PaCO2), with a resultant decrease in arterial oxygen tension (PaO2) in patients that are breathing room air [211]. Pure μ‐opioid receptor agonists [212,213] result in more significant depressant effects than partial [214] or mixed opioid agonists [215]. The magnitude of respiratory depression is directly correlated with the degree of sedation and/or analgesia produced [216]. Opioid overdose may limit the respiratory center’s response to profound hypoxia and hypercapnia, thus requiring full or partial reversal with administration of naloxone or butorphanol, respectively [217–220]. While effects are considered mild in healthy individuals, with PaCO2 and PaO2 often remaining within clinically acceptable limits, opioids may pose more significant risks in patients with respiratory compromise [221]. Mild reductions in PaO2 are a result of alveolar hypoventilation [212]. Elevations of PaCO2 are related to reductions in respiratory rate more so than decreased minute volume; however, elevations in respiratory rate (e.g., panting) may be observed [211]. Despite the latter, PaCO2 still remains elevated [211]. Opioid‐induced apnea is characterized by arrest of the respiratory cycle during the expiratory phase (i.e., impaired respiratory rate control) rather than from a profound decrease in tidal volume [222–224]. Both peripheral and central mechanisms associated with μ‐opioid receptor agonist stimulation may impair gastrointestinal motility or promote ileus. μ‐Opioid receptors are present in the enteric nervous system and are distributed in the stomach (corpus and antrum), duodenum, jejunum, ileum, and proximal and distal colon [225]. Stimulation of these opioid receptors suppresses neuronal excitability via inhibition of Ca2+ channels, membrane hyperpolarization, and decreased cyclic adenosine monophosphate and protein kinase A activity [225]. The net effect is decreased excitatory neurotransmitter release (e.g., acetylcholine, vasoactive intestinal polypeptide, and substance P) from acetylcholine‐ or substance P‐containing neurons [226,227]. Clinical effects lead to reductions in gastrointestinal motility, coordination, secretions, gastric emptying, and propulsive activity, as well as increases in pyloric and anal sphincter tone and constipation [225–227]. The effects of opioids on the large intestine are considered biphasic with initial stimulation of colonic motility leading to defecation shortly after administration followed by decreased colonic propulsive motility and secretions [64,187]. The route of administration may impact the magnitude of opioid‐induced gastrointestinal effects. In horses, IV administration of morphine has been reported to result in more significant gastrointestinal stasis when compared to epidural morphine administration [228,229]. Figure 23.3 Excitatory connections within the respiratory control center and opioid effects. Tonic chemodrive (green solid arrows) is the main excitatory drive to the medullary rhythm generator. A large component of chemodrive is routed through the Parabrachial Nucleus/Kölliker‐Fuse Complex (PBN/KF) to phase‐switching neurons in the preBötzinger Complex and determines respiratory rate (dark blue solid arrow). Phasic inputs from the preBötzinger Complex activate inspiratory (I) and expiratory (E) premotor and motoneurons (blue dotted arrows). Respiratory motor output (tidal volume) depends on direct projections from the retrotrapezoid nucleus (RTN) to preBötzinger Complex neurons, premotor neurons, and motoneurons. The corticolimbic system contributes tonic drive to the medullary rhythm generator (light blue solid arrows). Not shown: direct projections from the motor cortex to phrenic motoneurons as a pathway to override automatic rhythm; projections from the hypothalamus and cerebellum to the medullary raphe, which may contribute to the state‐dependency of respiratory activity. Opioid effects: Parabrachial Nucleus/Kölliker‐Fuse Complex activity was depressed in all studies and at all opioid concentrations. Opioid‐induced depression was also shown for the preBötzinger Complex, nucleus tractus solitarii (NTS), and the medullary raphe. Opioid‐induced sedation suggests depression of forebrain inputs. Premotor neurons are only directly depressed at very high opioid concentrations. Inset: neuronal subtypes constituting the core of respiratory rhythm generation. Through mutual excitation, network activity of pre‐inspiratory neurons (pre‐I) in the preBötzinger Complex results in activation of early inspiratory (early‐I) neurons (green arrows). Activity of these neurons is terminated through inhibition by postinspiratory neurons (post‐I), which themselves are inhibited by early‐I neurons during the inspiratory phase (red circles). Phasic excitation is relayed to inspiratory (I) and expiratory (E) premotor neurons. Source: Palkovic et al. [210], The American Physiological Society. Opioid‐induced vomiting is a result of δ‐opioid receptor stimulation within the chemoreceptor trigger zone (CTZ) outside the blood–brain barrier [230], a circumventricular organ located on the floor of the fourth ventricle in the postrema medulla [231]. Opioids with lower lipid solubility, such as hydromorphone, morphine, and meperidine, initially stimulate δ‐opioid receptors through fenestrated capillaries in the CTZ prior to crossing the blood–brain barrier where all opioids act at the medulla to inhibit the vomiting center (i.e., antiemetic effect) [230]. Thus, subsequent doses of opioid analgesic are less likely to result in vomiting compared to the initial dose [232]. Opioids that are highly lipophilic (i.e., fentanyl) penetrate the blood–brain barrier more rapidly and may inhibit vomiting [230,231]. Higher doses and/or concentrations of less lipophilic opioids (i.e., morphine and hydromorphone) may provide a more pronounced antiemetic effect [230,233]. For example, one study reported the incidences of morphine‐associated vomiting in dogs administered IV doses of 0.3, 1, and 2 mg/kg were 100%, 60%, and 0%, respectively [230]. SC and IM routes of administration of morphine and/or hydromorphone result in higher incidences of vomiting when compared to the IV route in dogs [62,234,235]. Morphine may induce a similar or slightly increased frequency of vomiting compared to hydromorphone in dogs [234,236]. In cats, vomiting may occur regardless of route, but usually less often following IV administration. For example, vomiting has been reported in approximately 83% (5/6 cats) [98], 40% (4/10 cats) [237], and 0% (0/6 cats) [238] to 86% (6/7 cats) [239] of cats administered hydromorphone via the SC, IM, and IV routes, respectively. Methadone and oxymorphone generally produce fewer vomiting episodes when administered by similar routes compared to hydromorphone or morphine [240]. Vomiting may still be observed with methadone when administered via the OTM route to dogs [241]. In a study involving healthy dogs, meperidine (8.8 mg/kg IM) resulted in no episodes of vomiting, while morphine (0.66 mg/kg IM) resulted in a 50% incidence of vomiting [242]. Several studies have reported ways to reduce opioid‐induced vomiting (Box 23.3) which include the use of acupuncture, maropitant, or acepromazine prior to hydromorphone or morphine administration [243–245]. Opioid administration has been reported to promote gastroesophageal reflux (GER) in anesthetized dogs [242,243,248,250,251]. Opioids potentiate GER via delayed gastric emptying, decreased lower esophageal sphincter tone, and reductions in gastroesophageal pressure [252–254]. Similar studies evaluating the effects of opioids on GER in anesthetized cats have not been performed; however, GER does occur in cats administered inhalant anesthetics [255]. Further discussion pertaining to GER frequency and species differences in dogs versus cats is beyond the scope of this chapter. The effects of opioids on GER may be dose related. Morphine administered IM at 0.22 and 1.1 mg/kg resulted in a GER frequency of 50% and 60%, respectively, compared to 27% in isoflurane‐anesthetized dogs that received a placebo [250]. Morphine combined with common premedication agents (e.g., dexmedetomidine, acepromazine, or midazolam) produced GER in 35–45% of inhalant‐anesthetized dogs [256]. Meperidine, when administered IM, is associated with a lower incidence of GER when compared to IM morphine in anesthetized dogs (8/20 or 40% versus 11/20 or 55%), respectively [242]. The incidence of GER with hydromorphone was reported to be approximately 30–69% in anesthetized dogs [243,251]. Butorphanol may in fact result in the highest incidence of GER among opioids, but this is difficult to determine as it has not been studied as a sole agent. One study reported the incidence of GER in dogs receiving butorphanol with either dexmedetomidine or acepromazine to be 53.3% and 66.7%, respectively [256]. Maropitant has not been shown to be effective in reducing GER associated with opioids in dogs [243]. Esomeprazole (1 mg/kg IV) with cisapride (1 mg/kg IV) administered 12–18 h and 1–1.5 h before anesthetic induction can reduce the incidence of reflux events when compared to placebo (11% versus 38%, respectively) in isoflurane‐anesthetized dogs administered SC hydromorphone for premedication and a fentanyl constant rate infusion (CRI) [257]. Metoclopramide administered at a high dose (bolus loading dose of 1.0 mg/kg IV, followed by CRI at 1.0 mg/kg/h) was associated with a 54% reduction in relative risk of developing GER in isoflurane‐anesthetized dogs administered morphine as premedication [258]. Lower doses (0.4 mg/kg IV loading dose followed by 0.3 mg/kg/h) did not significantly affect the incidence of opioid‐induced GER in a similar population [258]. In general, opioids have minimal adverse effects on cerebral blood flow (CBF), intracranial pressure (ICP), and cerebral metabolic oxygen consumption in humans, dogs, and cats [259–263]. Average CBF, oxygen extraction, and oxygen consumption were not significantly affected following morphine administration in cats [259]. However, regional changes may exist in areas with dense populations of opioid receptors [259]. Decreased CBF and oxygen extraction were noted in the hypothalamus, and decreased oxygen extraction and consumption were noted in the thalamic region following IV morphine administration in cats [259]. Indices of cerebral perfusion were significantly decreased at 30 min following morphine administration when compared to baseline in the right frontal cortex, whereas the left parietal cortex and subcortical region showed significantly increased perfusion indices at 30 min post‐morphine. No significant differences were noted for the other regions or at other time points [264]. Several studies have reported a decrease in CBF by up to 70% following systemic opioid administration in dogs [264–267]. Administration of morphine (1 mg/kg IV) also reduces spinal cord blood flow (SCBF) by similar amounts. Cerebral changes may be a result of decreases in metabolic oxygen demand and consumption as well as cerebral metabolic rate for glucose [265,266]. Morphine injected directly into the subarachnoid space was reported to have no effect on CBF and SCBF in dogs, which may indicate that the effects of opioids on cerebral hemodynamics are a result of their interaction with opioid receptors at supraspinal sites [265]. In cats, both decreased CBF [268] and no change in CBF [259] have been reported following opioid administration. Contrary to these findings, a study in cats found that CBF was significantly increased for approximately 20 min following sufentanil at doses of 10–200 µg/kg. Following this period, CBF gradually decreased below baseline levels at 60 min post‐administration [269]. Increases in CBF appear to be a result of decreases in cerebrovascular resistance [269] and, based on these findings, sufentanil should be used with some degree of caution in patients with acute increases in ICP. However, an alternative may be remifentanil as it has been reported to be an effective and safe analgesic in human patients with traumatic brain injury [261]. While there is conflicting evidence regarding the effects of opioids on ICP, most animal studies suggest that their impact is minimal [266,269]. Remifentanil administered at 0.05 to 1.0 μg/kg followed by a CRI at 0.03–0.26 μg/kg/min decreased ICP with minimal effects on cerebral perfusion pressure (CPP) in human subjects [270]. Remifentanil and alfentanil both reduced ICP during low‐ (0.5 and 1.6 μg/kg/min, respectively) and high‐dose (1 and 3.2 μg/kg/min, respectively) infusions in dogs anesthetized with 1.0% end‐tidal isoflurane and 50% nitrous oxide in oxygen [267]. However, increases in ICP post‐opioid infusion did occur during the recovery period. Sufentanil administration was reported to have little effect on ICP in adequately ventilated normal dogs and in dogs with elevated baseline ICP [271]. The use of fentanyl, sufentanil, and morphine at sedative doses in human subjects was well tolerated and did not result in changes in ICP. However, in another study, both fentanyl and sufentanil increased ICP in human head trauma patients [272]. Morphine may negatively impact recovery of neurologic function following acute spinal cord injury (SCI) (Fig. 23.4). The mechanisms mediating these effects can be classified as neuronal‐ or glial‐mediated. Morphine’s agonist effects at the κ‐opioid receptor inhibit locomotor function by inducing central sensitization, enhancing excitatory amino acid levels and augmenting N‐methyl‐D‐aspartate (NMDA) receptor‐mediated neurotoxicity through reduced glutamate reuptake, and negatively impacting spinal plasticity [273]. Opioids have also been reported to enhance immune cell trafficking (e.g., increased glial cell expression of Toll‐like receptors) and chemokine/cytokine activity, which can further increase pain and the development of neuronal apoptosis [273]. Several studies have evaluated the effects of morphine following SCI in rats [273–275]. Repeated morphine administration in rats significantly decreased the expression of neurons and glia following SCI when compared to vehicle controls [273,275]. The administration of morphine to rats also increased the size of the SCI lesion, delayed recovery of locomotor function post‐SCI, and contributed to the development of opioid‐induced hyperalgesia with subjects displaying increased reactivity to innocuous mechanical stimulation [273–275]. A one‐time administration of morphine within the acute phase following SCI (< 24 h) also contributed to the development of neuropathic pain at three weeks post‐injury [274]. To the authors’ knowledge, only one study has evaluated the effects of fentanyl administration in dogs undergoing thoracolumbar spinal surgery for intervertebral disk herniation [276]. In this study, fentanyl had little impact on the recovery characteristics in dogs [276]; however, this could be attributed to the lack of κ‐opioid receptor activity associated with fentanyl. Therefore, fentanyl could be a superior alternative to morphine in cases with SCI. Figure 23.4 A. Representative images (4×) are shown depicting neuronal (NeuN and NF) expression in one‐half of a spinal section, at the center of the lesion, for each treatment group of rats. Sham subjects are shown in the upper images; contused subjects in the lower images; panels on the left were treated with vehicle for seven days; panels on the right were treated with seven days of intravenous morphine. The contusion injury significantly decreased NeuN and NF expression at the center of the lesion, but when morphine was administered to the subjects with spinal cord injury (SCI) for seven days, there were virtually no neurons remaining at the injury site. B. There was a significant effect of SCI on NeuN expression at the center and rostral to the injury site, with less NeuN expression in the contused subjects. Morphine‐treated subjects with SCI had significantly less NeuN expression, at the center and rostral to the injury site, than both sham control groups. n = 3; **p < 0.05. Source: Hook et al. [275], with permission from Mary Ann Liebert, Inc. Opioid analgesics have a dose‐dependent effect on postoperative urinary retention [277,278], and can lead to increased bladder filling volume, increased micturition cycle duration, or a complete loss of the voiding phase via reduced coordination between external urethral sphincter activity and detrusor contractions [279]. μ‐Opioid receptor agonists are responsible for decreasing the force of detrusor contractions and the sensation of fullness via decreasing afferent inputs from the urinary bladder to the spinal cord [280]. Naloxone or methylnaltrexone have both been shown to reverse these effects [281]. Opioids can also decrease electrolyte excretion and promote antidiuresis via μ‐opioid receptor activation. In contrast to the μ‐opioid receptor effects, κ‐opioid receptor activation produces diuresis via suppressed release of arginine vasopressin (antidiuretic hormone) from the hypothalamus [282]. Epidural administration of opioid analgesics has been associated with the development of urinary retention in dogs [283–286] and cats [287,288]. Debate persists as to whether it occurs at a high enough incidence to determine its clinical relevance in these species [286,289], but it has been reported to be as high as 18% in dogs receiving epidural opioids during total hip replacement surgery [288,290]. Currently, data regarding the incidence of postoperative urinary retention after systemic opioid administration in other companion animal species are lacking [291]. When administered epidurally, methadone may have an opposite effect on the detrusor muscle by increasing its tone in dogs when compared to morphine [292]. Buprenorphine has been considered for epidural administration in veterinary species [113,293], as it may result in a lower incidence of urinary retention when compared to morphine [294]. However, data in humans suggest that urinary retention can occur with both full and partial μ‐opioid receptor agonists [295–297]. Lastly, administration of opioids at the lumbosacral level is associated with a higher incidence of urinary retention compared to opioids administered at the thoracic level [280]. Butorphanol, methadone, hydrocodone, codeine, and morphine are effective antitussive agents against pathologically and experimentally induced cough in the dog [298,299]. The duration of antitussive effects associated with morphine and butorphanol are longer than that of codeine, lasting approximately 4 h when administered as compounded oral formulations [299]. Tramadol also has antitussive properties in cats [300]. Naloxone inhibits the antitussive effects of morphine, butorphanol, and codeine but not dextromethorphan [299]. The antitussive effects of opioids are mediated predominantly by μ‐ and κ‐opioid receptors [301]. More recent evidence also suggests a potential role of δ‐opioid receptor antagonism in the antitussive effects of this class of drugs [302]. Opioid‐induced hyperthermia has been reported in cats and can occur following the administration of hydromorphone, buprenorphine, butorphanol, meperidine, morphine, and transdermal fentanyl patches [303–307]. Opioid‐induced hyperthermia (> 39.4 °C or 103 °F) is often self‐limiting (e.g., < 4 h) [305] with body temperatures in the range of 40–41.1 °C (104–106 °F) [307]. However, temperatures exceeding 42.2 °C (108 °F) have been reported [303] and, in these situations, treatment with IV naloxone may be required. High‐concentration buprenorphine administered at 0.24 mg/kg SC may predispose cats to greater risk of hyperthermia compared to morphine administered at 0.1 mg/kg SC [307]. Studies in rats suggest that opioids alter thermoregulation by raising the temperature set point that is controlled by the hypothalamus [308]. Horses may also exhibit transient mild increases in body temperature following administration of high‐dose hydromorphone (0.08 mg/kg), but not after 0.02 and 0.04 mg/kg [79], and after transdermal fentanyl application [309]. However, clinically relevant changes in body temperature with opioid administration in horses do not routinely occur [310–312]. In contrast to the effects of opioids on feline thermoregulation, canine subjects may experience hypothermia following opioid administration [313–315]. It has been postulated that the mechanism associated with hypothermia following opioid administration in dogs is due to lowering of the thermoregulatory set point [315]. Other species have been investigated, and the effects of opioids on body temperature appear to be dependent on dose, route, and the opioid administered [316]. The administration of IV morphine can cause sustained miosis in dogs; however, topical ocular administration does not appear to produce similar effects. Morphine has excitatory effects on pupilloconstrictor neurons in the visceral nuclei of the oculomotor nuclear complex in subcortical regions causing pupillary constriction [317]. More recent findings in humans administered fentanyl suggest that opioids also may induce miosis by blocking the inhibitory input into the pupilloconstrictor nucleus, resulting in reduced pupillary reflex dilation [318]. In cats, mice, and rats, morphine results in mydriasis via inhibition of the preganglionic parasympathetic nucleus (central component) and disruption of the release of acetylcholine from parasympathetic fibers of the ciliary nerves of the iris (peripheral component) [319]. Opioids exhibit a broad effect on the body’s immune system and in general inhibit cell‐mediated and humoral immunity as well as interact with inflammatory cytokines including interleukin (IL)‐1, IL‐4, IL‐6, and tumor necrosis factor [320–323]. Stimulation of μ‐opioid receptors by morphine and fentanyl decreases the expression of Toll‐like receptors on macrophages and suppresses natural killer (NK) cell function, respectively [324–326]. Morphine also may exhibit effects on tumor cells, modulating cell cycle, apoptosis, and angiogenesis (Fig. 23.5). Immune‐mediated responses and the effects of opioids on cancer cells are variable depending on the opioid and patient‐related factors. This variation, along with the limited clinical trials in companion animals and contradictory conclusions indicating both positive and negative effects on cancer development and metastasis, makes it difficult to definitively determine clinical implications. Figure 23.5 Mechanism of action of morphine on the tumor cell, modulating cell cycle, apoptosis, and angiogenesis. Source: Szczepaniak et al. [327], Springer Nature, CCBY 4.0, public domain. The impact of opioids on inhalant anesthetic minimum alveolar concentration (MAC) requirements depends on the type and dose of opioid and the species. In general, opioid agonists reduce MAC via an indirect effect on neurons associated with modulation within the dorsal horn of the spinal cord in response to a noxious stimulus [328]. A thorough review on opioid MAC‐sparing effects in dogs is available elsewhere [329]
23
Opioids
Introduction
History of opioid use in veterinary practice
Scheduling
Opioid crisis in North America: veterinary shopping, diversion, and opioid misuse and abuse in veterinary practice
Outcome
2014
2015
2016
2017
2018
2019
Number of patients with ≥ 1 veterinarian prescription
Any controlled substance class
479,755
538,996
577,161
567,021
502,291
448,541
Opioid analgesics
241,391
274,935
290,809
275,612
229,485
184,364
Opioid cough‐and‐cold medications
49,164
59,902
72,907
82,865
90,786
101,749
Benzodiazepines
199,151
215,884
225,762
219,831
191,707
171,140
Number of patients linked to potential vet‐shopping behavior
Any controlled substance classb
935
1711
2192
2325
2520
2875
Opioid analgesics
401
662
868
850
815
733
Opioid cough‐and‐cold medications
150
453
677
839
1041
1348
Benzodiazepines
185
274
335
375
381
440
Prevalence (%) of potential vet‐shopping behavior
Any controlled substance class
0.19
0.32
0.38
0.41
0.50
0.64
Opioid analgesics
0.17
0.24
0.30
0.31
0.36
0.40
Opioid cough‐and‐cold medications
0.31
0.76
0.93
1.01
1.15
1.32
Benzodiazepines
0.09
0.13
0.15
0.17
0.20
0.26
Pharmacology
Opioid receptors
Opioid receptor regulation
Opioid receptor localization
μ‐opioid receptor
κ‐opioid receptor
δ‐opioid receptor
Opioid receptor–opioid drug interactions
Pharmacokinetics
Absorption
Opioid
Route
Dose (mg/kg)
t½ (hours)
Vdss (L/kg)
Cl (mL/min/kg)
F (%)
Reference
Dog
Buprenorphineb
IV or SC
0.12
16.05
6.36
5.7
131
[59]
Butorphanol
IM or SC
0.5
1.62
7.96
57.5
[60]
Fentanyl
IV
0.01
0.75 ± 0.14
4.94 ± 0.86
77.9 ± 22.3
[61]
Fentanyl
IV + CRI
0.01 + 0.01/h for 1 h
0.5 ± 0.4
4.77 ± 0.25
61.9 ± 4.6
[61]
Fentanyl
IV + CRI
0.01 + 0.01/h for 3 h
0.86 ± 0.59
4.38 ± 0.39
46.9 ± 7.5
[61]
Fentanyl
IV + CRI
0.01 + 0.01/h for 4 h
0.69 ± 0.54
4.04 ± 0.26
47.9 ± 16.4
[61]
Hydromorphonec
IV
0.1
0.63 (0.50–0.66)
4.1 (3.3–5.5)
112 (83.7–135.4)
[62]
Hydromorphonec
IV
0.5
1.03 (0.70–1.44)
4.7 (3.8–5.3)
55.1 (45–85)
[62]
Hydromorphonec
SC
0.1
0.67 (0.61–0.80)
3.1 (3–3.6)
58.6 (44–74.2)
185
[62]
Hydromorphonec
SC
0.5
1.06 (1.04–1.21)
3.3 (3.2–4.1)
35.3 (33.2–40.4)
164
[62]
Meperidine
IV
2
0.61 ± 0.01
3.14 ± 0.58
77 ± 8.28
[63]
Morphine
IV
0.5
1.58 (1.09–2.2)
7.2 (5.9–9.0)
85.2 (71.6–99.2)
[64]
Morphine
IM
1
1.36 (0.97–2.23)
6.8 (2.1–7.2)
91.2 (83.1–94.6)
119 (57.9–161)
[64]
Morphine
Rectal
2
1.1 (0.66–1.66)
6.1 (3.6–9.0)
88.4 (85.6–90.3)
16.5 (10.9–27.4)
[64]
Tapentadol
IV
50
0.86 ± 0.2
6.45 ± 0.44
87.8 ± 22.62
[65]
Tapentadol
PO
200
0.35 ± 0.15
35.07 ± 20.37
57.92 ± 13.52
[65]
Tramadol
IV
4
1.02 ± 0.2
1 ± 0.47
15.38 ± 7.67
[66]
Tramadol
IM
4
2.28 ± 0.85
0.29 ± 0.15
18.85 ± 2.43
92 ± 9
[66]
Tramadol
PO
10
1.1 (0.9–1.3)
23 (15.4–39.8)
245 (143.9–543.7)
[67]
Cat
Buprenorphine
IV
0.01
6.9 ± 2.9
7.1 ± 3.2
16.7 ± 6.2
[68]
Buprenorphine
IM
0.01
6.3 ± 2.2
8.9 ± 5.9
23.6 ± 12.6
[68]
Buprenorphineb
IV, SC, OTM
0.12–0.24
12.3
7.89
16.33
94 SC, 23.6 OTM
[69]
Butorphanol
IM
0.4
6.28 ± 2.77
7.6 ± 6.28
12.92 ± 5.04
[70]
Butorphanol
OTM
0.4
5.23 ± 1.72
15.63 ± 5.7
25.35 ± 6.55
37.16 ± 14.95
[70]
Fentanyl
IV
0.00445–0.01116
2.35 ± 0.57
2.56 ± 0.32
19.83 ± 2.67
[71]
Meperidine
IM
5
3.6 ± 2.0
5.2 ± 2.1
20.1 ± 10.6
[68]
Morphine
IV
0.2
1.3 ± 0.3
2.6 ± 1.3
24.1 ± 10.3
[68]
Morphine
IM
0.2
1.6 ± 0.1
1.7 ± 0.8
13.9 ± 4.0
[68]
Remifentanil
IV CRI for 5 min
0.001
0.29 (0.09–15.34)
7.63 (2.28–76.04)
766 (408–1,473)
[72]
Tapentadol
IV
5
2.93 ± 0.86
8.79 ± 1.97
35.60 ± 7.05
[73]
Tapentadol
IM
5
2.28 ± 0.85
7.53 ± 2.95
37.85 ± 5.68
93.93 ± 9.91
[73]
Tapentadol
SC
5
2.05 ± 0.6
7.06 ± 2.10
40.13 ± 9.97
90.01 ± 6.52
[73]
Tramadol
IV
2
2.23 ± 0.3
1.6 ± 0.1
20.8 ± 3.2
[74]
Tramadol
PO
5.2
3.4 ± 0.13
3.7 ± 1.2
21.1 ± 3.0
93 ± 7
[74]
Horse
Buprenorphine
IV
0.005
3.58 ± 3.92
3.01 ± 1.69
7.97 ± 5.16
[75]
Buprenorphine
IM
0.005
4.24 ± 1.04
65.22 ± 16.74
[75]
Buprenorphineb
SC
0.0025
3.41 ± 0.93
5.23 ± 0.78
18.3 ± 3.2
[76]
Buprenorphineb
SC
0.005
4.77 ± 1.79
7.06 ± 2.87
17.18 ± 2.78
[76]
Butorphanol
IV
0.1
5.9 ± 1.5
1.4 ± 0.3
11.5 ± 2.5
[77]
Codeine
PO
0.3
1.84 (1.00–3.90)
[78]
Codeine
PO
0.6
1.94 (1.06–4.59)
[78]
Codeine
PO
1.2
2.60 (1.66–5.32)
[78]
Hydromorphone
IV
0.04
0.57 ± 0.21
1.46 ± 0.32
74.0 ± 10.5
[79]
Hydromorphone
IV
0.04
0.32 ± 0.15
1.13 ± 0.31
79.0 ± 12.9
[80]
Hydromorphone
IM
0.04
0.45 ± 0.15
155–200
[80]
Hydromorphone
IV
0.08
0.69 ± 0.54
2.24 ± 0.95
92.7 ± 13.9
[79]
Morphine
IV
0.2
6.43 (4.11–10.2)
7.96 (4.75–10.5)
34.5 (26.8–39.6)
[81]
Morphine
IV
0.2
5.17 (3.22–19.4)
6.72 (4.36–11.9)
32.8 (26.4–40.8)
[78]
Morphine
IM
0.1
1.48
4.49
34.9
[82]
Tramadol
IV
2
1.37 ± 0.17
2.17 ± 0.52
26 ± 3
[83]
Tramadol
IM
2
1.53 ± 0.23
111 ± 39
[83]
Cattle
Butorphanol
IV
0.25
82
4.18 ± 1.15
34.6 ± 7.7
[84]
Butorphanol
IV
0.25
82
4.18 ± 1.15
34.6 ± 7.7
[84]
Fentanyl
IV
0.005
12.7 (7.5–35.1)
24.8 (15.8–58.8)
33.3 (25.1–47.0)
[85]
Morphine
IV
0.5
1.18 ± 1.07
4.7 ± 3.0
37.3 ± 13.65
[86]
Morphine
IM
0.5
2.12 ± 0.82
[86]
Nalbuphine
IV
0.4
0.68 (0.53–0.79)
6.8 (4.9–8.9)
114 (104.3–129.7)
[87]
Sheep
Buprenorphine
IV
0.01
1.75 (1.07–31.0)
8.04 (1.05–49.3)
56.5 (4.17–100.33)
[88]
Fentanyl
IV
0.0025
3.08 (2.2–3.36)
8.86 (5.55–15.04)
60.33 (41.83–89.83)
[89]
Morphine
IV CRI for 10 min
1
1.98 ± 0.30
6.38 ± 0.9
34.3 ± 8.5
[90]
Oxycodone
IV
0.1
0.64 (0.33–4.4)
1.8 (1.5–4.7)
5.2 (4.6–6.2)
[91]
Tramadol
IV
4
0.67 ± 0.42
3.27 ± 1.92
74.43 ± 24.5
[92]
Tramadol
IV
6
0.57 ± 0.12
4.29 ± 0.75
78.87 ± 58.48
[92]
Goat
Butorphanol
IV
0.1
1.87 ± 1.49
1.27 ± 0.63
96 ± 24
[93]
Butorphanol
IM
0.1
2.75 ± 1.93
82 ± 41
[93]
Fentanyl
IV
0.0025
1.2 ± 0.78
1.51 ± 0.39
34.83 ± 10.33
[94]
Morphine
IV
0.4
2.67 ± 0.82
9.64 ± 6.18
121.25 ± 38.53
[95]
Morphine
IM
0.4
1.73 ± 0.30
144.6 ± 50.5
[95]
Morphine
SC
0.4
1.63 ± 0.17
131.9 ± 61.3
[95]
Tapentadol
IV
5
3.22 ± 3.27
4.39 ± 1.94
74.15 ± 18.9
[96]
Tapentadol
IM
5
1.29 ± 0.54
87.8 ± 35.6
[96]
Tramadol
IV
1
0.94 ± 0.34
2.48 ± 0.58
36.33 ± 3.83
[97]
Tramadol
PO
2
2.67 ± 0.54
30 ± 7
[97]
Distribution
Metabolism
Excretion
Opioid tolerance, dependence, withdrawal, and opioid‐induced hyperalgesia
Species
Route
Dose, frequency/duration
Comments
Reference
Dog
Not reported
3 mg/kg, daily
At 21 days post‐administration sedation no longer observed
At 10 days post‐administration vomiting no longer observed
[124]
SC
0.5 mg/kg and 2.5 mg/kg twice daily or infused at 1–5 mg/kg/day
Dogs developed opioid tolerance within 8 days of administration
[125]
Cat
IV
1–4 mg/kg/day
Minimal tolerance observed at 7 days of administration
[126]
SC
1–2 mg/kg/day divided into morning and evening doses
Tolerance was developed by 24–30 days. Tolerance was overcome via increasing the morphine dose
[127]
Clinical pharmacology
Analgesia
Sedation, euphoria, and dysphoria
Cardiovascular system
Respiratory system
Gastrointestinal system
Nervous system
Renal system
Antitussive effects
Thermoregulatory effects
Ophthalmic effects
Immune system effects and effects on cancer metastasis
Effects on inhalant anesthetic minimum alveolar concentration
Dogs
Stay updated, free articles. Join our Telegram channel
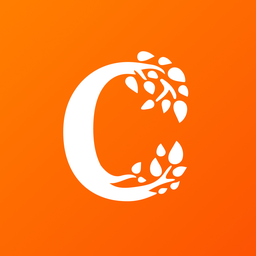
Full access? Get Clinical Tree
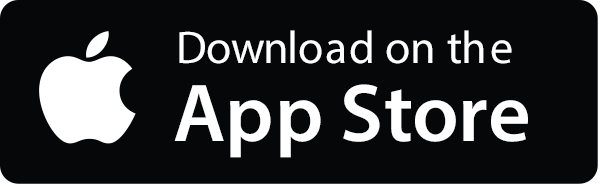
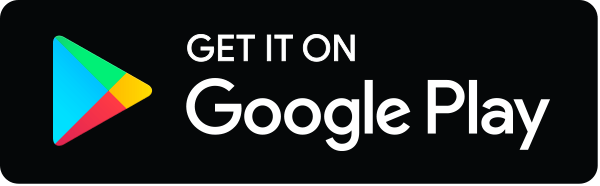