Vaidehi V. Paranjape1 and Luisito S. Pablo2 1 Department of Small Animal Clinical Sciences, Virginia-Maryland College of Veterinary Medicine, Virginia Tech University, Blacksburg, Virginia, USA 2 Department of Comparative, Diagnostic, and Population Medicine, College of Veterinary Medicine, University of Florida, Gainesville, Florida, USA Patients with ophthalmic diseases or injuries often need general anesthesia for surgery or diagnosis. The anesthetic goals for ophthalmic patients to ensure favorable outcomes should focus on: (1) regulating intraocular dynamics; (2) preventing and treating the oculocardiac reflex; (3) providing adequate analgesia; (4) managing systemic effects of ophthalmic medications; and (5) anticipating surgical outcomes that can influence anesthetic care (e.g., hemorrhage). Detailed knowledge of ocular anatomy and physiology is also important for improving patient outcomes. Knowledge of ocular anatomy (Figs. 67.1 and 67.2) enables an anesthesiologist to understand the array of ocular surgeries, as well as gain mastery of local and regional anesthesia performed for these cases. The orbit is a bony fossa that encompasses the eye, associated muscles, nerves, and vasculature. It protects the eye as well as separates it from the cranial cavity. All vertebrate orbits are of two types: (1) the enclosed orbit, which is completely surrounded by bone (e.g., horses, sheep, cattle, and goats), or (2) the open or incomplete orbit, which is only partially surrounded by bone (e.g., dogs, cats, and pigs) [1,2]. The bones that form the orbit in dogs, cats, cattle, and sheep are the lacrimal, zygomatic, frontal, sphenoid, palatine, and maxillary. In horses, goats, and pigs, there is no involvement of the maxillary bone. Instead, the temporal bone and the ethmoid bone contribute to the orbit in horses and pigs, respectively. The orbital foramen is differently structured in the horse and is therefore referred to as the “orbital fissure” in this species. In cattle and pigs, the orbital fissure and foramen rotundum are typically fused to form the foramen orbitorotundum [1,2]. The orbital fascia is a thin, tough connective tissue layer that lines all the structures within the orbit. This fascia encompasses three anatomic structures: the periorbita, Tenon’s capsule, and the extraocular muscles (EOM). The orbital fat fills up the spaces between these structures and acts as a protective cushion for the eye. The EOMs suspend the globe in the orbit and provide ocular mobility. The seven orbital muscles that control the movement of the globe are the dorsal rectus, ventral rectus, medial rectus, lateral rectus, dorsal oblique, ventral oblique, and retractor oculi (Table 67.1) [1,2]. The retractor oculi muscle is absent in birds and snakes [3]. The eyelids are two musculo‐fibrous folds of the skin. The space between the upper and lower eyelids is called the “palpebral fissure.” Closure of the palpebral fissure is achieved by contraction of the orbicularis oculi muscle. Opening of the eyelids is mediated by the relaxation of the orbicularis oculi muscle as well as contraction of the levator palpebrae superioris muscle (Table 67.1) [2,4]. Figure 67.1 Ocular anatomy. Source: Dr. Vaidehi Paranjape, with permission. Figure 67.2 The nasolacrimal system. Source: Dr. Vaidehi Paranjape, with permission. The conjunctiva is the clear thin mucous membrane that lines the inner aspect of the eyelids, the nictitating membrane, and the exposed sclera. The nictitating membrane is a thin translucent sheet of ocular tissue situated ventromedial to the globe, which is actively retracted across the cornea to provide protection and lubrication. It is well developed in domestic animals, while in higher primates, it is a vestigial structure. In most domestic animals, the movement of the nictitating membrane occurs due to contraction of the retractor oculi muscle. However, in cats, since the retractor oculi muscle is less developed, adjacent smooth muscle bundles may contribute to its movements [2]. Table 67.1 Function and innervation of eyelid muscles and extraocular muscles. Source: Adapted from Meekins et al. [2]. CN, cranial nerve. The globe is made of three tunics that surround the transparent ocular media, which are the aqueous humor, the lens, and the vitreous humor. The outermost fibrous tunic is further divided into the cornea and sclera. The avascular cornea protects the internal structures of the eye, contributes to the refractive power of the eye, and forms a retinal image via focusing of light rays. The sclera is a tough, opaque layer filled with a dense network of collagen and elastic fibers. There is also a cartilaginous component seen in fish, lizards, chelonians, certain amphibians, and birds [3]. It is the supporting wall of the globe and helps maintain the structure of the eyeball and protects it from injury. The thick middle layer is the vascular tunic, commonly referred to as the “uvea” or “uveal tract.” It is interposed between the retina and the sclera and is heavily pigmented and vascularized. The iris, ciliary body, and choroid are three subparts of the uvea. The pigmented iris controls the quantity of light entering the eye through the pupil, as well as influences the pupil size. The heavily pigmented ciliary body provides nourishment and removes waste from the cornea and lens and participates in lens accommodation. The choroid is composed of blood vessels and pigmented support tissues, and it nourishes the outer retinal layers. The innermost layer is the nervous tunic, consisting of the retina and optic nerve. The ten‐layered neurosensory retina is connected to the brain by the optic nerve and the optic tracts. The rods and cones (i.e., retinal photoreceptors) transform light stimuli from the external environment into nerve impulses and transmit these signals to the brain where they are converted to visual images. The optic nerve extends from the globe to the optic chiasm, and forms optic tracts. The transparent, avascular, and crystalline lens focuses light rays on the sensory retina, providing optimal focusing power for visual clarity. In domestic animals, branches of the external carotid artery (i.e., the internal maxillary and external ophthalmic arteries) form the primary blood supply to the eye and its adnexa. The internal ophthalmic artery is a smaller artery that arises from the rostral cerebral artery at the level of the optic chiasm, providing blood supply for the optic nerve. The long and short posterior ciliary arteries are derived from the external ophthalmic artery, and they supply most of the blood to the anterior segment of the eye, including the retina and the choroid. The retinal arteries are derived from the short posterior ciliary arteries as they pass through the sclera at the periphery of the optic nerve [2,4]. There is significant variation within domestic animals with respect to the venous drainage of the eye. In dogs, the two main venous channels are the supraorbital and the inferior orbital veins. The supraorbital vein further unloads into two prominent veins, the orbital vein entering intracranially, and the internal maxillary vein connecting with the external jugular vein. The cat has an external rete, which is drained by a large ophthalmic vein connecting to the external jugular vein. Also, the supraorbital vein drains into the facial vein, finally emptying into the internal jugular vein. In horses, the principal ocular venous channels are the ophthalmic, orbital, supraorbital, and reflex veins [2,4]. The eye and its adnexa are innervated by the optic, oculomotor, trochlear, trigeminal, abducens, and facial nerves (Table 67.2, Fig. 67.3). Assessment of palpebral and the corneal reflexes is considered standard monitoring during general anesthesia in animals to characterize the anesthetic depth. The palpebral reflex is tested by lightly touching the lateral and medial canthi, which results in eyelid closure. The afferent pathway is via the ophthalmic and maxillary branches of the trigeminal nerve, for medial and lateral stimulation, respectively. The efferent arm is the facial nerve. The corneal reflex is assessed by touching the cornea with a wisp of cotton wool or a swab tip or a drop of sterile water, which should result in retraction of the globe and eyelid closure. The afferent arm of the corneal reflex is mediated by the ophthalmic branch of the trigeminal nerve, and the efferent arm is mediated by the abducens nerve (globe retraction) and facial nerve (eyelid closure) [9]. Table 67.2 Cranial nerves innervating the eye and its adnexa. Source: Compiled from Murphy et al. [4]; Herrera et al. [6]; Shin et al. [7]; Joffe and Gay [8]. CN, cranial nerve. There are intricate ocular physiologic processes, which can be impacted during the perioperative period. Aqueous humor (AH) is a transparent, colorless liquid that fills the anterior and posterior chambers including the pupil. It nourishes the cornea and the lens, removes waste products, and maintains the intraocular pressure and globe shape. The mean AH turnover rate among several species is approximately 1–2% of the anterior chamber volume per minute, with cats having a lower turnover rate (0.7%/min) when compared to dogs and humans [10]. Two‐thirds of AH is produced in the posterior segment of the ciliary body via active mechanisms such as the sodium pump, carbonic anhydrase‐catalyzed reaction of CO2 hydration, and the cytochrome oxidase systems. These active processes lead to a higher AH osmotic pressure than the plasma, and this disparity promotes a continuous production of AH. The remaining one‐third is passively formed at the iris capillaries by diffusion of lipid‐soluble substances moving down a concentration gradient, as well as ultrafiltration from plasma in response to an osmotic gradient or hydrostatic pressure. Figure 67.3 Innervation to the eye. Source: Miller [5]. The AH exits the eye through two pathways. In the conventional or trabecular route, aqueous fluid passes from the posterior chamber, through the pupillary aperture into the anterior chamber. From there it flows through the trabecular meshwork into the angular aqueous plexus, finally draining into the episcleral veins, conjunctival veins, and vortex venous system that join the systemic venous circulation. The majority of the AH (i.e., ~50% in horses, 85% in dogs, and 97% in cats) leaves the eye via this route [11]. The unconventional or uveoscleral pathway excludes the trabecular meshwork. The AH bathes the iris and the ciliary muscle to reach the supraciliary space or the suprachoroidal space. From here, it seeps through the sclera and is absorbed into the orbital vasculature. Uveoscleral outflow drains the remainder of the AH (i.e., ~15% in dogs [12] and 3% in cats [13]). Horses appear to have a higher dependency on this pathway [14]. The vitreous humor (VH) is a transparent elastic hydrogel that comprises a portion of the clear ocular media, and accounts for up to two‐thirds of globe volume. It occupies the space between the lens and sensory retina and maintains structural integrity of the posterior portion of the globe. It is part of the optical pathway through which light passes as it travels to the retina. The VH is 99% water, and the remaining 1% is a network of polygonal, hydrated fibrils consisting of type II collagen and hyaluronic acid [11]. The collagen provides plasticity and hyaluronic acid contributes to the viscoelasticity of the VH. The pressure exerted by ocular contents against the globe is termed “intraocular pressure” (IOP). In the healthy eye, IOP is generated by a delicate balance between rates of AH inflow and outflow, keeping it at a steady state when the rates are equivalent. The most important influence on AH formation is the difference in osmotic pressure between AH and plasma [15]. The relationship is illustrated as: where K is coefficient of outflow, OPaq is osmotic pressure of AH, OPpl is osmotic pressure of plasma, and CP is capillary pressure. This equation explains why mannitol, due to its hypertonicity, can lower IOP by influencing AH production. The normal IOP range in dogs, cats, and rabbits is 15–20 mmHg, while in cows and horses, IOP ranges from 20–30 mmHg and 17–28 mmHg, respectively [11]. The IOP can be theoretically determined by the Goldmann equation: where Q represents the aqueous inflow (in μL/min), U represents the uveoscleral outflow (in μL/min), C represents conventional aqueous outflow facility through the trabecular meshwork (μL/min/mmHg), and EVP is the episcleral venous pressure [11]. The primary factors impacting IOP are: (1) pressure on the eyeball; (2) muscle tone of EOM and orbicularis oculi muscle; (3) AH production and drainage, (4) corneal and scleral rigidity; (5) volume of intraocular contents; and (6) episcleral venous pressure (Table 67.3). Direct pressure applied on the globe during physical restraint, ocular examinations, opening of mouth for intubation, passing of an Elizabethan collar over the head, and globe manipulation during surgery can dramatically elevate IOP. Table 67.3 Factors impacting intraocular pressure. Source: Compiled from Hendrix et al. [11]; Cunningham and Barry [17]; Komaromy et al. [24]; Kelly and Farrell [25]. aExpected to decrease IOP but conflicting data available in the literature bExpected to increase IOP but conflicting data available in the literature cResponse is dosage dependent IOP, intraocular pressure; BP, blood pressure; CVP, central venous pressure; AH, aqueous humor; NMBA, neuromuscular blocking agent. The central nervous system (CNS) impacts IOP directly through neurogenic control of extraocular muscle tone by central diencephalic centers, and indirectly via hormonal and hemodynamic effects. Increased tone or contracture of EOM and the orbicularis oculi muscle can markedly increase IOP, which is observed during rapid blinking, forced eyelid closure, inadequate plane of anesthesia, and with drugs like ketamine and succinylcholine. In contrast, general anesthesia lowers IOP partly by depressing the central control centers. The rate of AH formation by the ciliary epithelium is influenced by sympathetic and parasympathetic innervation, as well as humoral mechanisms. Topical β‐adrenergic receptor antagonists (e.g., timolol) and α2‐adrenergic receptor agonists (e.g., brimonidine) may lower IOP by decreasing AH production. During sleep, AH formation decreases by about 50%. Further, depending on whether animals are nocturnal or diurnal, variations in AH production and IOP may follow a circadian rhythm [16]. Acute break down of the blood–aqueous barrier during ocular trauma can lead to plasma leakage into the anterior chamber, thus mimicking AH overproduction and increasing IOP. Outflow of AH can be impacted by increases in jugular venous pressure and central venous pressure (CVP). Collection of debris or blood in the anterior chamber and abnormalities of the iridocorneal angle can cause impeded AH outflow and glaucoma. The most significant factor controlling AH outflow is the diameter of Fontana spaces (i.e., spaces within the trabecular meshwork), as explained by the Hagen–Poiseuille law [17]: where A is the volume of AH outflow per unit of time, r is the radius of Fontana spaces, Piop is the IOP, Pv is the venous pressure, η is the viscosity, and L is the length of Fontana spaces. During pupillary dilation, the Fontana space narrows, resistance to flow increases, and a marked rise in IOP is seen. Hence, during glaucoma, patients are treated with topical miotics. Resistance offered by the sclera and cornea to a change in intraocular volume is termed “ocular rigidity.” Changes in intraocular volume will affect ocular rigidity. Also, scleral and corneal compliance decreases with age, increasing IOP in older animals. When ocular tumors or systemic malignancies that spread to the eye occur, IOP can escalate. Ocular circulation autoregulates in response to changes in the ocular perfusion pressure via metabolic and myogenic mechanisms. Hyperoxia, hypocapnia, and alkalosis induce vasoconstriction in the retinal and choroidal arterioles, thus reducing IOP. The opposite effect on IOP occurs with hypoxia, hypercapnia, and acidosis [17]. Disorders associated with substantially decreased blood flow to the eye (e.g., dehydration, hypovolemic shock, and cardiogenic shock) can result in lower mean ocular perfusion pressure, retinal ischemia, and decreased IOP. During chronic arterial hypertension, IOP returns to normal due to adaptive mechanisms triggered by compression of choroidal vessels. This feedback mechanism reduces the total ocular blood volume, with IOP staying fairly constant. However, acute increases in blood pressure may lead to transient elevations in IOP. Episcleral venous pressure (EVP) is the “backpressure” created by the episcleral veins of the conventional pathway near the angular aqueous plexus. The pressure gradient between the anterior chamber and the episcleral veins has a significant effect on the rate of aqueous flow through this route and constitutes approximately 50–75% of the resistance that determines IOP. Increases in jugular venous pressure and CVP can augment EVP and IOP. This can happen during jugular compression, the Trendelenburg position [18], and other postural changes as seen in dogs [19], cats [20], and horses [21–24]. Variation in the pupil size is regulated by a balance between the iris sphincter (constrictor) and iris dilator muscle groups. The iris sphincter is a ring of smooth muscle cells connected by gap junctions and is located within the pupillary border. The oculomotor nerve provides parasympathetic innervation to the sphincter muscle, thus causing miosis. In contrast, the iris dilator is a myoepithelial layer extending from the iris root, where muscle fibers are also interconnected with gap junctions. The dilator muscle is innervated by sympathetic nerves and is responsible for mydriasis. This sympathetic activity in the dilator muscle is mediated by a combination of α‐ and β‐adrenergic receptors [11]. Prostaglandins are involved in maintaining the muscle tone of iris sphincter muscles and can cause miosis. Thus, prostaglandin analogs like latanoprost possess IOP‐lowering activity and are used for glaucoma treatment in dogs [26]. Pupillary diameter is a primary factor affecting the success of cataract surgery and the likelihood of complications. Hence, sympathomimetics and anticholinergics can be useful mydriatics during cataract surgeries. The exception is the avian eye, where the constrictor and dilator muscles are mainly striated and, as such, the pupil is not affected by traditional mydriatics [27]. The precorneal tear film (PTF) is a complex blended three‐layered structure comprised of an outer lipid layer, middle aqueous layer, and deep mucin layer. The PTF serves multiple functions such as: (1) maintaining an optically uniform cornea; (2) removing debris and foreign material from the ocular surface; (3) lubricating the conjunctiva and cornea; (4) providing nutrients and oxygen to the avascular cornea; (5) controlling local bacterial flora; and (6) preserving ocular immunity. Fibers from the ophthalmic division of the trigeminal nerve (i.e., lacrimal nerve), facial nerve, pterygopalatine ganglion, and sympathetic fibers from the carotid plexus provide innervation to the lacrimal gland. Parasympathetic innervation of the lacrimal gland results in reflex tearing [28]. In animals, the aqueous portion of the tear film is routinely measured by the Schirmer tear test. Schirmer I does not need topical anesthesia and evaluates both basal and reflex tearing. Schirmer II is carried out with topical anesthesia and measures only basal tearing. Results of studies in humans [29], dogs [30–32], cats [33], and horses [34] report significant reductions in tear flow during general anesthesia. Dryness in the corneal epithelium predisposes patients to painful postanesthetic abrasions or corneal ulcers. These sequelae can be prevented with periodic ocular lubrication during the anesthetic period. Larger dogs demonstrate greater wetting per minute than smaller dogs [35]. Additionally, canine neonates have lower tear production than adults [36]. Hence, special care needs to be taken in these patients presented for anesthesia. There are two primary ocular barriers, the blood–aqueous barrier (BAB) and the blood–retinal barrier (BRB). In combination, these maintain homeostasis by protecting the eye and preventing entry of lethal substances into internal ocular components. The BAB is the anterior barrier of the eye and is composed of capillary endothelial cells of the iris and non‐pigmented epithelial cells of the ciliary body. This barrier can be altered during ocular inflammation, intraocular surgery, trauma, or vascular diseases. The BRB is the posterior barrier comprised of retinal pigment epithelium and retinal vascular endothelium equipped with non‐leaky tight junctions. This restricts intercellular permeation of drugs after systemic and periocular application to the retina. Although these barriers offer protective functions, they can also impede entrance of many drugs into the eye, diminishing therapeutic efficacy [37]. Variations in the BRB may lead to the development of retinal diseases such as diabetic retinopathy and age‐related macular degeneration. The oculocardiac reflex (OCR) (i.e., Aschner reflex or trigeminovagal reflex) induces reflexive slowing of the heart rate and cardiac dysrhythmias such as atrioventricular blockade, ectopic atrial rhythm, junctional rhythm, multifocal ventricular premature contractions, ventricular bigeminy, ventricular ectopy, asystole, and ventricular fibrillation [38]. This reflex is more commonly encountered in pediatric human patients during strabismus surgery [39] as compared to adults. The OCR has been reported in dogs [40], cats [41], horses [42], rabbits [40], and birds [43]. The incidence of the OCR may be increased by hypercapnia [44], hypoxemia [38], a light plane of anesthesia [39], and drugs such as short‐acting opioids (e.g., sufentanil and remifentanil) [45] and dexmedetomidine [46]. The triggers for the OCR include external pressure on the eyeball, ocular manipulation, traction on the EOM, conjunctiva and orbital structures, orbital injections, retrobulbar block, ocular trauma, ocular pain, ocular hematomas, and facial trauma [7,8,47]. As a fatigable reflex, the intensity of the OCR may diminish with repeated stimulation. Upon activation of stretch receptors in the eye, short and long ciliary nerves conduct sensory impulses to the ciliary ganglion. From there, the impulses are transported via the ophthalmic division of the trigeminal nerve to the Gasserian ganglion and trigeminal nucleus. This afferent pathway continues along short internuncial fibers in the reticular formation to connect with the efferent limb. Signals are returned via the visceral motor nucleus of the vagus nerve to the sinoatrial node in the heart [38] (Fig. 67.4). There are also oculo‐respiratory [7] and oculo‐emetic [48] reflexes characterized by a similar afferent pathway, but which carry different efferent signals. Immediate management of the OCR includes (1) communication with the surgeon and temporary cessation of surgical manipulation until heart rate and rhythm normalize; (2) confirmation of optimum ventilation, oxygenation, and anesthetic depth; (3) administration of a systemic anticholinergic, if warranted, due to significant or persistent bradycardia; (4) possible infiltration of local anesthetic around the ocular muscles (e.g., rectus muscles), which may abolish the afferent pathway; and (5) initiation of cardiopulmonary resuscitation in the event of asystole. Various maneuvers to abolish or obtund the OCR have been suggested but warrant further investigation. In dogs undergoing enucleation, preoperative administration of an anticholinergic drug was not associated with a lower prevalence of the OCR [50]; thus preemptive anticholinerigc use should be determined on a case‐by‐case basis depending on patient needs. Preoperative retrobulbar anesthesia can blunt the afferent pathway and help reduce incidence of OCR in dogs [50] and horses [51]. Use of a nondepolarizing muscle relaxant may also attenuate this reflex by reducing extraocular muscle tone [52]. Figure 67.4 The oculocardiac reflex pathway. Source: Tobin and Weaver [49]. Ophthalmic medications (Table 67.4) are routinely administered either topically or systemically for ocular procedures. Topical drugs have the potential for systemic absorption. This creates possibilities for drug interactions and anesthetic complications. Smaller patients, neonates, and pediatrics may be at a higher risk. Systemic absorption occurs either via conjunctival vasculature or from nasal mucosa after draining through the nasolacrimal duct [53]. Effective communication with the ophthalmologist prior to the anesthetic event regarding frequency of drug administration or need for additional diagnostics to identify existing systemic effects (e.g., hypokalemia seen with acetazolamide) can prevent related adverse effects during the perioperative period. Table 67.4 Ophthalmic medications used in patients. IOP, intraocular pressure; IV, intravenous. Patients undergoing eye surgery benefit from sedatives and anxiolytics to provide comfort and anxiolysis during examination, restraint, intravenous (IV) catheter placement, anesthetic recovery, and hospital stays. An ideal induction agent provides a rapid onset of action, avoiding the risk of regurgitation and IOP fluctuations. The proposed mechanism of action of anesthetics in reducing IOP may involve a direct effect on central diencephalic control centers, lowered arterial blood pressure (e.g., inhalant anesthetics), reduction of aqueous production, facilitation of aqueous drainage, or relaxation of extraocular muscle tone [17]. Acepromazine causes miosis in dogs when given alone or in combination with hydromorphone [82,83]. A significant decrease in tear production with acepromazine has also been reported in dogs [84,85], rabbits [86], and cats [87]. A 20% decline in IOP values was seen in horses following intravenous administration [88]. Similar findings were reported in dogs [82,83]. Due to its antiemetic properties, vomiting or gagging may be prevented in patients that are at risk of globe rupture or vitreous prolapse. Since rough and abrupt anesthetic recoveries have a potential for causing postoperative eye trauma and bleeding, acepromazine may promote smooth, slow, and quiet recoveries that can benefit ophthalmic patients. Benzodiazepines either maintain or decrease IOP in dogs [89,90] and cats [91], as well as blunt the increase in IOP induced by ketamine [92]. However, contradictory findings have also suggested that diazepam alone or with ketamine can increase IOP in healthy dogs [93,94]. Midazolam reduces etomidate dose requirements and improves intubation conditions; however, this combination does not affect IOP in dogs [95]. Propofol–atracurium anesthesia in dogs elevates IOP, but this effect is dampened by diazepam. Endotracheal intubation‐induced increases in IOP are not prevented by diazepam [96]. Intramuscular diazepam is observed to cause no significant change in tear production in rabbits [86]. The combination of tiletamine–zolazepam (Telazol®) up to 20 mg/kg IV induced no clinically significant changes in IOP in healthy dogs [97]. Similarly, in clinically normal cats, 10 mg/kg Telazol® intranasally or intramuscularly (IM) produced no change in IOP [98]. In glaucomatous cat eyes, there was no effect on IOP with 2 mg/kg IM Telazol®; however, 4 mg/kg IM increased IOP by 8%. Zolazepam alone up to 2 mg/kg IM reduced IOP in normal and glaucomatous cat eyes [99]. An increase in IOP values post Telazol® was also detected in capuchin monkeys [100]. Xylazine induces mydriasis in cats and rats, possibly due to central inhibition of parasympathetic tone or stimulation of α2‐adrenergic mechanisms in the iris [101]. Moreover, xylazine decreases IOP in rabbits, cats, and monkeys by suppressing sympathetic neuronal function and reducing aqueous flow [102]. In dogs treated with 8 mg/kg IM xylazine, significant reductions in IOP were observed, but no change in pupil size was seen [103]. In rabbits, subcutaneous ketamine–xylazine decreased IOP by nearly 5 mmHg and blunted the isoflurane‐associated increase in IOP seen in this study [104]. In healthy horses, IV xylazine caused a dose‐dependent decline in IOP [88,105,106]. Use of xylazine in rats and mice has been associated with acute reversible lens opacity [107]. Xylazine has also been shown to decrease tear flow in cats [87,108] and dogs [109,110], but not in horses [34]. Topical medetomidine lowered IOP in cats and rabbits and produced concurrent mydriasis [111]. This finding suggests distribution of α2‐adrenergic receptors in the eye that may regulate IOP. In contradiction, however, miosis was noted with IV medetomidine administration in non‐glaucomatous dogs, without any influence on the IOP [112]. Medetomidine at 80 μg/kg IM caused a significant decline in IOP in healthy dogs [103], while a 100 μg/kg IM dose did not change IOP in healthy cats [113]. Medetomidine–butorphanol IV increased IOP for about 20 min in dogs [114]. Systemic medetomidine has consistently been shown to decrease tear flow in dogs [115,116], cats [117], pigs [118], and chimpanzees [119]. In healthy horses, IV detomidine at 10 and 20 μg/kg decreased IOP, suggesting this drug is a safe sedative when performing ocular procedures in this species [105,120]. Similar findings were reported with IV romifidine in horses [121,122] and buffaloes [123]. Dexmedetomidine IV significantly lowered IOP and caused miosis in dogs [82,124]. Another canine study recommended that dogs sedated with dexmedetomidine–butorphanol receive a tear substitute [125]. Intrathecal dexmedetomidine led to dose‐dependent antinociception and mydriasis in conscious rats [126]. Dexmedetomidine alone or in combination with butorphanol, meperidine, methadone, nalbuphine, or tramadol resulted in decreased IOP for 120 min in dogs [127]. After topical application, dexmedetomidine lowered IOP in normal as well as glaucomatous rabbit eyes [128]. The potential for α2‐adrenergic receptor agonists to cause nausea and vomiting in small animals must be considered when planning sedation or anesthesia in patients that may be adversely affected by IOP fluctuations. There have been few studies across species investigating the influence of pentobarbital and thiopental on ocular dynamics. Pentobarbital significantly lowered IOP as compared to urethane, chloralose–urethane, and halothane, and depressed the formation of AH via its direct effect on AH outflow facility in cats [129]. A dose‐dependent decline in IOP by 20–50% was reported in both normal and glaucomatous eyes in cats administered pentobarbital [99]. A similar IOP‐lowering effect with thiopental is observed in dogs [130]. In horses, thiopental significantly lowered IOP as compared to guaifenesin–ketamine and propofol [131]. While in humans, thiopental not only decreased IOP, but also helped negate the increase in IOP induced by succinylcholine [132]. Propofol attenuates an increase in IOP in anesthetized humans and lowers IOP as compared to inhalants [133]. The effects of propofol on IOP and end‐tidal carbon dioxide (PETCO2) have been studied in dogs and no decrease in IOP was observed, likely due to the opposing effects of increased PETCO2 on IOP [134]. In several canine studies, propofol‐associated increases in IOP are reported similar to horses [131], sometimes even abolishing the IOP‐lowering effect of premedication [94,135,136]. Mean IOP was elevated after propofol administration in non‐premedicated dogs without glaucoma and remained steady in non‐premedicated dogs with glaucoma. In glaucoma‐affected eyes of premedicated dogs, IOP declined after premedication and propofol administration [137]. A limited number of canine studies have compared the ocular effects of propofol and alfaxalone, with no differences in IOP observed between the two groups [135,138]. However, alfaxalone, but not propofol, significantly lowered tear production [138]. In sheep, alfaxalone does not alter IOP but causes marked miosis [139]. Associated myoclonus, intact ocular reflexes, nystagmus, blepharospasm, and open palpebrae have traditionally made ketamine a less desirable choice for ophthalmic patients [17]. Previous work has suggested that ketamine may increase IOP. Postulated reasons for this include increases in EOM tone or changes in AH production and outflow, or an increase in CVP. Since an increase in IOP could be detrimental during glaucoma or open globe injury, ketamine’s use for ocular surgeries remains controversial [17,25]. In cats, IM ketamine (12.5 mg/kg and 25 mg/kg) caused dose‐dependent increases in IOP in glaucomatous eyes, while a 10% rise was seen over awake IOP values in normal eyes [99]. Ketamine alone or in combination with diazepam in dogs and horses caused clinically significant increases in IOP [90,93,94,131]. Similarly, in rabbits, IM ketamine–diazepam or ketamine–acepromazine combinations elevated IOP [140]. Other studies have called into question the assertion that ketamine is not appropriate in ophthalmic patients due to its effects on IOP. In dogs, 15 mg/kg ketamine with 0.2 mg/kg midazolam IV resulted in no change in IOP [92]. Also, ketamine–xylazine IM and ketamine–diazepam IV for induction maintained IOP in dogs [141]. In rabbits, monkeys, and sheep, IOP declined significantly with ketamine used alone or combined with xylazine or diazepam [104,142–145]. Even Telazol® has been reported to maintain steady IOP in cats, dogs, and monkeys [97,98,143]. Etomidate causes a significant decrease in IOP in humans which, to some extent, is unexpected in light of the associated myoclonus and arterial blood pressure stability that are characteristic of this drug. A fall in IOP by 61% was seen along with mydriasis followed by miosis and low frequency pendular nystagmus in human patients [146–148]. In dogs premedicated with IV butorphanol and induced with etomidate–midazolam or etomidate–lidocaine, no change in IOP or evidence of myoclonus was observed [95]. Etomidate–midazolam in another canine study produced clinically important miosis and increases in IOP [149]. Interestingly, in rabbits, topical etomidate produced no change in pupillary diameter and light reflex, but did cause decreases in IOP, conjunctival chemosis, and corneal epithelial edema [147]. In the 1960s, methoxyflurane became a popular choice over halothane for ophthalmic anesthesia due to: (1) better cardiovascular stability; (2) centrally fixed eyes during light surgical anesthesia and ocular hypotonia; (3) lower incidence of bleeding; (4) smooth and slow emergence; and (5) reduced need for postoperative analgesia and sedation. However, the average decrease in IOP was greater for halothane (18%) as compared to methoxyflurane (6%) [150]. The introduction of isoflurane provided comparable decreases in IOP to halothane, but with lower arrhythmogenicity, better cardiac index, and significantly lower incidence of postoperative nausea and vomiting [151–153]. Currently, isoflurane, sevoflurane, and desflurane are the volatile anesthetics used in veterinary species. All three agents provide comparable reductions in IOP during ocular surgeries [154
67
Ophthalmic Patients
Introduction
Ocular anatomy
Orbit
Ocular adnexa
Muscle name
Function
Innervation
Dorsal rectus
Moves globe upward
Oculomotor (CN III)
Ventral rectus
Moves globe downward
Oculomotor (CN III)
Medial rectus
Moves globe medially
Oculomotor (CN III)
Lateral rectus
Moves globe laterally
Abducens (CN VI)
Dorsal oblique
Pulls dorsal part of globe medially and ventrally
Trochlear (CN IV)
Ventral oblique
Pulls ventral part of globe medially and dorsally
Oculomotor (CN III)
Retractor oculi
Retraction of globe
Abducens (CN VI)
Levator palpebrae superioris
Elevates upper eyelid
Oculomotor (CN III)
Orbicularis oculi
Closure of palpebrae
Facial (CN VII)
Retractor anguli oculi
Lengthens palpebral fissure
Facial (CN VII)
Globe
Ocular circulation
Ocular innervation
Cranial nerve
Principal ocular functions
Optic (CN II)
Carries vision fibers from the retina and pupillomotor axons; responsible for the pupillary light reflex, dazzle reflex, and accommodation reflex
Oculomotor (CN III)
Provides primary general somatic efferent innervation to the eye muscles, and motor innervation to the upper eyelid, pupil, and lens
Supplies motor innervation to the dorsal rectus, medial rectus, ventral rectus, ventral oblique, and levator palpebrae superioris muscles
Contains parasympathetic preganglionic general visceral efferent axons that synapse in the ciliary ganglion; postganglionic fibers from the ciliary ganglion innervate the iris sphincter and the ciliary muscles
Trochlear (CN IV)
Innervates the dorsal oblique muscle, thus controlling abduction and intorsion of the eye
Trigeminal (CN V)
Trigeminal ganglion contains cell bodies of the general somatic afferent neurons of this nerve; distal to the ganglion, it divides into its three main branches:
Facilitates the oculorespiratory cardiac reflex in response to manual pressure on the eyeball
Plays a vital role in the ocular pain pathways
Abducens (CN VI)
Responsible for the extraocular motor functions of the eye
Supplies motor innervation to the lateral rectus and retractor bulbi muscles
Facial (CN VII)
Supplies somatic efferent innervation to the muscles of the eyelids and parasympathetic innervation to the lacrimal gland
At level of the external acoustic meatus, the facial nerve continues as the auriculopalpebral nerve, originating near the zygomatic process of the temporal bone; branches of the auriculopalpebral nerve innervate the dorsal and ventral portions of the orbicularis oculi, retractor anguli oculi lateralis, and levator anguli oculi medialis
The zygomatic branch of the facial nerve further divides into the upper branch supplying the frontalis and upper eyelid orbicularis, whereas the lower branch supplies the lower eyelid orbicularis
Ocular physiology
Aqueous humor
Vitreous humor
Intraocular pressure
Pressure on the eyeball
Increase IOP
Decrease IOP
Increase or decrease IOP
Decreased or obstructed AH outflow
Increased AH production
Acute increase in BP
Hypercapnia
Hypoxemia
Acidosis
Laryngoscopy, endotracheal intubation
Direct pressure on the globe
Retrobulbar block
Jugular compression
Increase in CVP
Coughing, gagging, vomiting, sneezing, straining
Inadequate anesthetic depth
Head position lower than the body, Trendelenburg position, other postural changes
Succinylcholine
Atropine
Increased AH drainage
Decreased AH production
Hypocapnia
Hyperoxia
Alkalosis
Hypotension
Hypothermia
Barbiturates
α2‐Adrenergic agonists
β‐Adrenergic antagonists
Cholinergic agonists
Carbonic anhydrase inhibitors
Benzodiazepines
Acepromazine
Opioids
Nondepolarizing NMBAs
Inhalant anesthetics
Osmotic agents
Diurnal effect
Seasonal effect
Propofola
Alfaxalonea
Ketamineb
Etomidateb
Phenylephrinec
Epinephrinec
Muscle tone of extraocular and orbicularis oculi muscles
Aqueous humor production and drainage
Ocular rigidity
Volume of the intraocular contents
Episcleral venous pressure
Pupil size
Tear production and drainage
Ocular barriers
Oculocardiac reflex
Systemic effects of ophthalmic medications
Drugs
Route
Mechanism of action
Use
Systemic effects
Tropicamide, atropine, homatropine, cyclopentolate, scopalamine
Topical
Cholinergic receptor antagonist
Cause mydriasis and cycloplegia
Atropine: profuse salivation and occasional vomiting in dogs [54] and cats [55]; decreased intestinal motility, and abdominal pain in horses [56,57]
Phenylephrine, epinephrine
Topical
Direct acting sympathomimetic
Cause mydriasis and ocular vasoconstriction
Phenylephrine: hypertension and reflex bradycardia in dogs [58,59] and cats [60]
Epinephrine: hypertension, tachycardia, and dysrhythmias in humans [61]
Pilocarpine
Topical, oral
Cholinergic receptor agonist
Causes miosis
Vomiting, regurgitation, diarrhea, drooling, bradycardia, and weakness in dogs and cats [62]
Demecarium bromide
Topical
Carbamate parasympathomimetic
(acetylcholinesterase inhibitor)
Causes miosis
Suppression of systemic acetylcholinesterase levels, gastrointestinal disturbances in dogs [63]
Echothiophate iodide
Topical
Organophosphate parasympathomimetic (acetylcholinesterase inhibitor)
Causes miosis
Suppression of systemic acetylcholinesterase levels and possibility for organophosphate toxicity in dogs [63]; prolonged muscle paralysis from neuromuscular blockers, and bradycardia reported in humans [64]
Apraclonidine, brimonidine tartrate
Topical
α2‐Adrenergic receptor agonist
Reduce IOP
Apraclonidine: reduces heart rate, salivation, vomiting in cats and dogs [65,66]
Brimonidine tartrate: sedation, hypotension, bradycardia in dogs and cats [67,68]
Timolol, levobunolol, betaxolol, metipranolol, carteolol, nipradilol
Topical
β‐Adrenergic receptor antagonist
Reduce IOP
Timolol: reduces heart rate in dogs [69] and cats [70]; bronchoconstriction, and congestive heart failure in humans [71,72]
Levobunolol: decreases pulse rate in dogs [73]
Acetazolamide, methazolamide, dorzolamide, brinzolamide
Oral, topical
Carbonic anhydrase inhibitor
Reduce IOP
Acetazolamide: increased diuresis, gastrointestinal disturbances (anorexia, vomiting, and diarrhea), hypokalemia, and increased respiratory rate secondary to metabolic acidosis in small animals [74,75]
Mannitol
Glycerol
Hypertonic saline–hydroxyethyl starch
IV
Oral
IV
Hyperosmotic agent
Hyperosmotic agent
Hyperosmotic agent
Reduces IOP during acute crisis
Reduces IOP during acute crisis
Reduces IOP during acute crisis
Diuresis, increased CVP, increased serum osmolality, decreased urine specific gravity, and pulmonary edema in small animals [76,77]
Nausea, vomiting, hyperglycemia, glycosuria, diuresis, weight gain in dogs [76,78]
Volume expansion, changes in electrolytes in dogs [79]
Prednisolone, dexamethasone, hydrocortisone
Topical, systemic
Inhibition of cyclo‐oxygenase and lipoxygenase pathways
Resolve ocular inflammation
Exacerbate ocular infections, delay corneal healing, cataract formation, ocular hypertension, adrenal suppression, hyperglycemia, lipid keratopathy, exophthalmos [80,81]
Effects of anesthetic drugs and adjuvants on ocular physiology
Sedatives
Phenothiazines
Benzodiazepines
α 2‐Adrenergic receptor agonists
Injectable anesthetics
Barbiturates
Propofol and alfaxalone
Dissociatives
Etomidate
Inhalant anesthetics
Stay updated, free articles. Join our Telegram channel
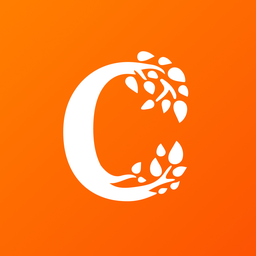
Full access? Get Clinical Tree
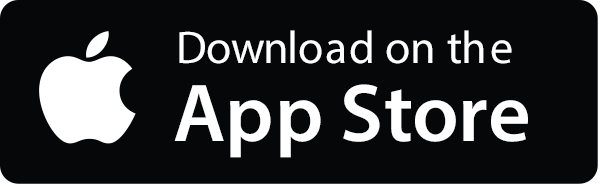
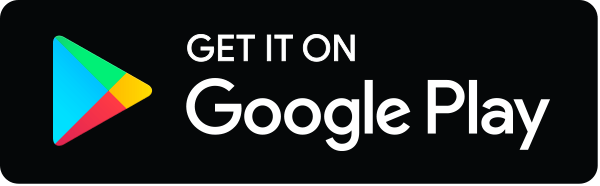