Revised from 6th edition of Veterinary Ophthalmology, Chapter 7: Sections 1, 2, 3, 4, and 5 – Clinical Pharmacology and Therapeutics, by Alain Regnier, Alison Clode, Erin M. Scott, Amy Rankin, Ian P. Herring, and Caryn E. Plummer In veterinary ophthalmic pharmacology and therapeutics, those drugs of importance as primary front‐line drugs are presented for the treatment of ophthalmic diseases. Often by understanding the different disease processes, as well as the strengths and weaknesses of the selected drugs, critical windows for therapy can be identified for the maximum effects of these drugs. Critical factors in ocular pharmacology are reducing the significant barriers for drug entry at the corneal surface, the blood–aqueous barrier (BAB) at the ciliary body epithelium, and the blood–retinal barrier for the posterior segment. These barriers can also be changed in certain diseases, so investigations of drug delivery and pharmacokinetics must be conducted in both normal and diseased eyes. The fact that in the drug development process animals are used to understand the drug properties, and then drugs are tested in clinical trials in humans facilitates drug development and access in veterinary ophthalmology. Also, as a drug is exposed to multiple species, a greater appreciation of the drug’s effect and usefulness becomes evident. Future drug development will emphasize not only the drug’s effectiveness, but also its toxicity and their ability to target specific areas of a disease process more effectively. As compliance is a major problem for all drug therapy, frequency of drug administrations is an important limiting factor, especially for long‐term drug therapy and chronic eye diseases. Hence, dosage regimen and route of administration should also be adapted to clinical management of the patient in order to maximize owner compliance. The bulk of ophthalmic drugs are administered locally as drops or ointments. While this tends to limit systemic side effects, local administration has its own set of restrictions because the eye is a unique organ with several functional and structural protective mechanisms, such as blinking, permanent lacrimation, and lacrimal drainage, which are necessary to preserve visual acuity, but favor rapid removal of drugs topically applied to the ocular. For drugs periocularly or systemically administered, the sclera and the blood ocular barriers, which are critical for maintaining the delicate homoeostasis of the transparent media, act as major obstacles for the access of xenobiotics to intraocular target tissues. Increasing knowledge of drug absorption and disposition combined with advances in drug delivery system technology has provided newer approaches for optimal bioavailability, prolonged action, and improved safety of ocularly delivered drugs. Compared with drug delivery to other organs of the body, ocular drug delivery is a major challenge because the ocular barriers refer to anatomical and physiological ocular structures that have protective functions for maintaining ocular homeostasis and represent natural defense mechanisms against the entry of xenobiotics into the eye. For topically applied drugs, the corneal route has been assumed to be the major route of entry into the eye. The cornea consists of three primary layers, the epithelium, stroma, and endothelium, representing distinct barriers to absorption organized as an aqueous phase (stroma) sandwiched by two lipid layers (epithelium and endothelium). Drug passage through the corneal epithelium can occur both across the cells (transcellular route) and between the cells (paracellular route). The paracellular route is blocked by one type of specialized intercellular junction, the tight junction or zonula occludens characterized by multiple sites of fusion between the plasma membrane of adjoining cells, which completely surround and seal the superficial epithelial cells of the cornea to all but the smallest hydrophilic molecules. As a consequence, the transcellular route across the lipid cell membrane will contribute to the epithelial transfer of lipophilic drugs, while very low molecular weight hydrophilic (polar) compounds will diffuse through the intercellular space, which represents the aqueous pore pathways of the corneal epithelium. The corneal stroma with its 78% water content allows the free passage of compounds possessing high aqueous solubility and acts as a barrier to lipophilic molecules. The endothelium does not provide significant resistance to lipophilic and hydrophilic ophthalmic drugs. Penetration across the conjunctiva, and then sclera, contributes significantly to the intraocular penetration of certain topically applied drugs. The conjunctiva is more permeable (2–30 times higher) than the cornea through a significant paracellular route, which makes its permeability to molecules of varying physicochemical characteristics, such as beta‐blockers, hydrophilic macromolecules, and [3H] mannitol. The sclera may represent a barrier to intraocular transfer of either topically applied drugs that are absorbed via the conjunctiva/scleral route of entry or those that are injected periocularly. Scleral permeability was found to be approximately 10 times higher than that of the cornea with a direct relationship between the ability of a drug to penetrate sclera and both the thickness and total surface area of this tunic. Studies in rabbits suggest the molecular radius is a better predictor of scleral permeability than is the molecular weight. The primary route for solute transport through the sclera is by passive diffusion through the interfibrillar aqueous media of the gel‐like proteoglycans. Following systemic administration, penetration of drugs into the eye is limited by the endothelial cells of iridial and retinal vessels and epithelial cells of the ciliary body and retinal pigment epithelium (RPE). In these barriers, the paracellular route is blocked because the clefts between the endothelial or epithelial cells are sealed by impermeable tight junction complexes that prevent the entry of solutes into the ocular environment (i.e., aqueous humor [AH] and vitreous body). In the anterior segment of the eye, the permeabilities of the ciliary body and iris vasculature and epithelium are remarkably different (Figure 3.1). In the stroma of the ciliary processes, circulating macromolecules escape through the capillary walls but their transfer to the posterior chamber is blocked by the tight junctions that interconnect the apices of the nonpigmented ciliary epithelium. However, in the iris the endothelial cells of the vessels represent the other anatomical location of the BAB because they lack fenestrae and are joined by tight junctions, which prevents movement of macromolecules from the lumen of the iridial vessels into the iris stroma, and then into the anterior chamber. Figure 3.1 The BAB in the anterior segment consists of the endothelial cells of the iris blood vessels and the nonpigmented cell layer of the ciliary epithelium and their tight junctions. ABL, anterior border layer; NPL, nonpigmented layer of the ciliary epithelium; PE, posterior epithelium; and PL, pigmented layer of the ciliary epithelium. In the posterior segment, the two barriers to penetration include the endothelial cells of the retinal vessels (also referred to as the inner blood–retinal barrier) and the cells of the RPE (sometimes termed the outer blood–retinal barrier). The retinal vessels are nonfenestrated and have tight junctions, creating an obstruction to movement of substances from plasma into the retina and vitreous. The outer blood–retinal barrier is produced by the tight junctions between the RPE cells, so that substances leaking out from the extremely permeable capillaries of the choriocapillaris encounter this barrier of junctional complexes between RPE cells. Topical administration is the most common route of administration for ophthalmic drugs because of its advantages, including simplicity of application and convenience to reach both extra‐ and intraocular tissue targets (Figure 3.2). The pharmacokinetic profile of topically applied ophthalmic drugs is influenced by precorneal factors (i.e., lacrimation and drainage) and the specific characteristics of the formulation itself that can influence the amount of drug penetrating the eye. Eye drops represent the pharmaceutical formulations most widely used in veterinary ophthalmology and consist of solutions and suspensions. Ophthalmic solutions are formulations in which the drug is totally dissolved in a given solvent. Typically, they are low‐viscosity, aqueous solutions mixable with the aqueous tear film. Thus, the drug must be, at least to some degree, water soluble. To minimize irritation of the eye, ophthalmic solutions must ideally have an osmolality (tonicity) of about 300 mOsm/kg, which is the tonicity of normal tears. However, various studies have shown that the eye can tolerate solutions with an osmolality in the range of 200–600 mOsm/kg, or 0.2–2.0% in NaCl equivalents. To improve stability and sterility, ophthalmic solutions are formulated with appropriate vehicles that may contain buffers, organic or inorganic carriers, emulsifiers, and wetting agents. Most agents are well tolerated, cause little discomfort, and do not affect vision. When frequent applications of ophthalmic solutions are required in animals, especially horses (i.e., in the treatment of severe corneal infections), a continuous or intermit irrigation system, such as a subpalpebral lavage system or a nasolacrimal cannula, is necessary to provide frequent instillations with minimum handling. Figure 3.2 Disposition of ophthalmic drugs after instillation to the eye. Only a small portion of topically applied drug may reach the posterior segment. Pharmaceutical derivatives of low aqueous solubility, such as acetates and alcohols used as topical corticoids, require formulations as ophthalmic suspensions. A suspension consists of particles of active ingredient in a saturated aqueous vehicle that includes dispersing and suspending agents designed to be instilled into the eye. The drug particles contained in suspension must be less than 10 μm, uniform in size, and micronized to prevent irritation of the ocular surface. All multidose eye preparations must include a bacteriostatic preservative (i.e., benzalkonium chloride, benzethonium chloride, methylparaben, propylparaben, mercurial compounds, and thimerosal) to prevent or inhibit microbial growth during clinical use. Of primary concern with their frequent or prolonged use in humans is their potential toxicity to the ocular surface epithelium, disruption of tear film stability, and hypersensitivity reactions. As benzalkonium chloride is used in most antiglaucoma agents, it is presently assumed that the similar inflammatory ocular surface changes induced during long‐term treatment in humans directly influence the outcome of filtering surgery by increasing the risk of bleb encapsulation. Unpreserved eye drops are supplied in unit dose containers or multidose bottles, but the range is still limited, so they cannot be used in all cases. As indicated in Figure 3.3, an ophthalmic drug topically applied to the eye is distributed in three ways. It is drained by the nasolacrimal apparatus, may penetrate into the eye through the corneal and/or noncorneal routes, and is absorbed into the systemic circulation via the conjunctiva and nasopharynx. Figure 3.3 Topically applied medications can enter the systemic circulation though the conjunctival absorption, drainage via the nasolacrimal system, and absorption through the nasopharyngeal mucosa. After transcorneal penetration, minor routes of systemic drug transfer involve AH outflow and diffusion into the iris. When eye drops are administered to the ocular surface, they first mix with the tear film compartment, the volume of which is about 7–10 μl (with 1 μl covering the cornea and about 3–4 μl residing in each conjunctival sac), as estimated in humans and rabbits. The drop volume delivered by many ophthalmic dropper bottles is about 40 μl on average. Since the palpebral fissure is capable of holding only 25–30 μl of fluid, the volume of most ophthalmic drops largely exceeds the volume of the cul‐de‐sac, so complete retention of this drop volume is unlikely to occur. The tear volume and tear flow rate in horses have been reported to be 230 μl and 33 μl/min, respectively. The rate at which the instilled drug is eliminated from the ocular surface due to drainage by the nasolacrimal system is influenced by three main factors: the size of the drop delivered to the eye, the blinking frequency, and the tear flow dynamics. Within the cul‐de‐sac, the drainage rate of an instilled volume has been shown to be proportional to the volume of the drop that is above the normal lacrimal fluid volume. The larger the volume instilled, the more rapidly it is drained through the nasolacrimal system. As an instilled drop is removed in about 10 min, dosage guidelines therefore recommend that at least 10 min elapse between the instillation of drops of different medications. After topical ocular application, drugs may be absorbed into the inner eye through the corneal or conjunctival–scleral route. The rate and extent of absorption through one route or the other are dependent both on transport characteristics of the cornea, conjunctiva, and sclera and on the physicochemical properties of the drug itself. Transfer through the epithelium is the rate‐limiting step for absorption for hydrophilic compounds, whereas transfer through the stroma is rate limiting for lipophilic compounds. Thus, in order for an ophthalmic drug to penetrate the cornea, it must exhibit intermediate solubility characteristics, being soluble to some degree in both oil and water to penetrate the epithelium and stroma. For moderately lipophilic drugs such as timolol and dexamethasone, the corneal epithelium contributes 50% to the total resistance to transport, while the stroma and endothelium each contribute 25%. For hydrophilic drugs such as epinephrine and pilocarpine, the corneal epithelium contributes more to the total resistance. A very lipophilic drug, such as cyclosporine (CSA), will penetrate the epithelium readily, but the stroma will be rate determining. Conversely, very hydrophilic drugs such as gentamicin, tobramycin, prednisolone sodium phosphate, dexamethasone sodium phosphate, cromolyn, and idoxuridine will penetrate the epithelium slowly or not at all because the paracellular pathway predominates. In the case of ionizable drugs, the pH of the ophthalmic preparation and that of the tear fluid affect ocular drug penetration by influencing the ratio of the ionized (dissociated) and unionized (nondissociated) forms of the molecule in equilibrium. The degree of ionization of a drug in solution, and hence its capacity to diffuse across cellular barriers, is determined by its dissociation constant (pK a) and the solvent’s pH. Protein binding of topical drugs is known to occur in the precorneal tear film, rendering the bound fraction no longer available for absorption. As local inflammation increases tear protein content, drug loss by this route may gain significance during topical treatment. Hydrophilic drugs that penetrate poorly through the intact epithelium reach very high levels in the cornea when the epithelium is damaged or inflamed. In the case of a corneal ulcer, drug is further retained by the craterlike effect of irregular tissue margins. Experimentally, it has been shown that penetration into the cornea and anterior chamber of a topically applied hydrophilic antifungal agent is increased up to ninefold when 25–50% of the surface area of the corneal epithelium is removed. In the two last decades, studies have shown that the conjunctival–scleral absorption of ocularly applied drugs contributes to the ocular absorption known as noncorneal absorption. The noncorneal absorption route through conjunctiva and sclera is important mostly for very hydrophilic and large molecules that are not able to penetrate through the corneal barrier (examples include topical carbonic anhydrase inhibitors [CAIs]). Once the drug penetrates the conjunctiva, there are two different possibilities for it to reach the intraocular target tissues. Drug entry directly into the anterior chamber can result from lateral diffusion into the sclera and then the cornea, or it enters the scleral blood vessels that supply the uvea, particularly the anterior ciliary arteries, and deposits within the ciliary body. A large part from each drop applied to any eye is drained through the nasolacrimal system, where the vascularized lining of the nasolacrimal duct and nasopharyngeal mucosa is available for systemic absorption. Systemic drug absorption also results from uptake by fenestrated blood vessels located in the conjunctiva/episclera and must also be considered. This seems the case for the ophthalmic beta‐blockers, which show an extremely low drug concentration in plasma after corneal application compared to conjunctival and scleral applications. The significance of circulatory system access by topical agents is that these medications entering the systemic circulation through the nasolacrimal mucosa bypass the primary metabolism in the liver in this way. The administration of eye drops has therefore been compared with a slow intravenous injection, which can account for the systemic toxicity of some topically applied ophthalmic preparations such as beta‐blockers. After topical drug application in one eye, significant contralateral effects may sometimes be observed. It is generally postulated that drugs can enter the fellow eye by way of the systemic circulation after absorption across the conjunctiva of the treated eye or the epithelial lining of the nasolacrimal duct. Changes in intraocular pressure (IOP) in the contralateral eye have been noted with topical application of various antiglaucoma agents but have been particularly documented for beta‐blockers in humans and animals. Topical atropine in one eye in the dog can lower Schirmer tear test (STT) I levels in both eyes. Topical timolol in one eye of cats or dogs lowers IOP in treated and nontreated eyes. Since part of the instilled drugs is absorbed systemically, the technique to deliver drugs into systemic circulation via ocular route has been studied. The delivery of systemic insulin through the ocular route in healthy cats and dogs, and diabetic dogs indicates that systemic absorption of insulin may occur subsequently to topical ocular administration. Pharmacological effects after topical instillation of drugs indicate improvement of an adequate blood flow to the retina or optic nerve head with antiglaucoma agents. Significant intraocular distribution occurs after topical application of various drugs such as brimonidine, betaxolol, dorzolamide, nepafenac, and dexamethasone–cyclodextrin. Following intraocular penetration of topically applied drugs, their subsequent distribution and retention at the target site are critical to their therapeutic success. Those drugs that diffuse through the cornea enter the AH first and then are distributed to the iris–ciliary body, lens, and vitreous. The peak aqueous concentration occurs from 0.5 to 3 h after instillation, and was estimated in humans to be about a 150 000 dilution of the drop for a hydrophilic drug and a 1500 dilution for a lipophilic one. For topically applied drugs that penetrate the eye via the noncorneal route of entry, higher peak concentrations may be observed in the iris–ciliary body before the AH, because scleral penetration permits the drug to reach the target tissue first without entering the AH. Aqueous outflow is presumed to be the primary route of drug elimination from the eye, although loss of drug may occur by additional pathways (i.e., retinal blood flow) or processes such as metabolism and drug binding to distinctive tissues. Once drugs are absorbed into the anterior chamber, they are eliminated mostly by AH turnover, which was estimated to be 1.5–5 μl/min in humans and about 5 μl/min in dogs. In dogs, the clearance rate of marbofloxacin from AH (5 μl/min) was found to be very close to that reported for AH turnover. One established form of binding is the affinity of drugs to melanin present in different ocular tissues. The iris and ciliary body have a heavily pigmented layer of epithelial cells, which can influence the pharmacological effect of instilled ophthalmic drugs, because accumulation of drug in this pigmented tissue by melanin binding decreases the free drug concentration available for target tissues. The higher the amount of melanin in the eye, the smaller is the initial mydriatic effect of atropine. Topical pilocarpine accumulates up to 10 times more in the pigmented rabbit eyes than in the albino anterior uvea and its miotic effects are significantly prolonged in the pigmented rabbit eyes compared to albino ones. This effect also occurs in blue eyes of horses, dogs, and cats, but has not been quantified. Although many oxidoreductase, hydrolytic, and conjugating enzymes that exist in systemic tissues are expressed in various ocular tissues, little is known about the extent of local metabolism. Ocular drug metabolism is still evolving, and is being investigated in prodrugs and soft drug analogues. Esterase activity is the highest in the iris–ciliary body, followed by the cornea and then the AH. Depending on the nature of the drug, these enzymes contribute to either an inactivating or activating effect. Esterases are responsible for inactivating pilocarpine and idoxuridine. Drugs topically applied to the eye do not obey the classic pharmacokinetics based on systemic absorption, because unique factors affect drug absorption across the ocular surface membranes. As most of the instilled volume is rapidly lost from the preocular area, ophthalmic solutions exhibit a fast drug pulse delivery with an initially high concentration that rapidly declines to a concentration below the therapeutic range (Figure 3.4). The disappearance of a drug from ophthalmic solution follows first‐order kinetics in which the instilled drug available for target tissues declines exponentially as the medication is lost through the nasolacrimal system and is washed away by the tear turnover. It is generally considered that for ophthalmic drugs, less than 1% to no more than 10% of the dose topically applied enters the eye. Increasing the frequency of drug administrations can directly influence drug concentrations in the target ocular tissues. Because only a very small fraction of the drug applied to the eye can be absorbed into the inner eye, many schemes have been developed to improve ocular bioavailability of medications delivered to the eye. Subcutaneous abdominal pumps, lacrimal duct cannulation and external infusion pumps for delivery of topical pilocarpine as an antiglaucoma agent or artificial tears for dry eye have been reported in humans. Continuous delivery of irrigating fluid to the eye is used in humans for the treatment of acute chemical burn. The Morgan lens is a convenient device for continuous ocular irrigation in human patients. Continuous ocular treatment was also proposed for horses to reduce labor of treatment and improve its pharmacological effects; the feasibility and potential usefulness of a subconjunctivally implanted micro‐osmotic pump and a continuous infusion pump connected to a subpalpebral lavage system have been evaluated. The nonbiodegradable micro‐osmotic pumps implanted subconjunctivally were well tolerated by the horses and allowed effective delivery of atropine during the seven days they were in place. Subpalpebral systems in horses can also be used with intermittent drug administrations. They ensure drug delivery to the eye and reduce the difficulty associated with topical drug administrations in horses. Ointments can achieve prolonged delivery, but can blur vision. The thick, oleaginous bases of ophthalmic ointments are primarily mixtures of white petrolatum and mineral oil, with or without a water‐miscible agent such as lanolin. Hydrophilic drugs are dispersed in the base as particles, as in suspensions, while lipid‐soluble drugs are dissolved in the ointment base. Ophthalmic ointments must contain preservatives in order to prevent bacterial contamination during use, and effects of pH and tonicity should be controlled as previously discussed for ophthalmic solutions and suspensions. Drug–eye contact time can be improved in part by increasing the viscosity of the vehicle with the addition of methylcellulose, a hydrophilic polymer. These research efforts led to a significant body of literature demonstrating that these polymers can prolong precorneal residence time and improve ocular bioavailability of hydrophilic drugs. Experimentally, it was determined that the improvement in ocular drug delivery reached a maximum level at a viscosity of about 12–15 cps and that higher viscosity formulations caused ocular irritation with an increase in reflex tears and blinks leading to rapid elimination of the instilled drug. Figure 3.4 The differences between the drug levels between single eyedrop instillations, and an ophthalmic insert. The goal is to try and maintain drug therapeutic levels as high as possible. Applications of nanotechnology currently represent a very exciting field of research for development of new delivery drug systems to the eye. The main focus has been given to colloidal systems consisting of micro/nanoparticles, micro/nanoemulsions, nanosuspensions, and liposomes. Liposomes are vesicles (0.01–10 μm) composed of one or more phospholipid bilayers separated by aqueous compartments containing aqueous core, which have been widely exploited in ocular drug delivery. The potential use of nanoparticles as an ophthalmic drug delivery system has been extensively evaluated for various hydrophilic and hydrophobic drugs, including pilocarpine, amikacin, betaxolol, carteolol, CSA, and indomethacin, loaded into or on nanospheres of different polymers. Soft contact lenses used for the treatment of a variety of corneal diseases as a support bandage can also serve as nonerodible vehicles for progressive drug release in the tear pool. Soft contact lenses are typically composed of nonbiodegradable hydrophilic polymers, such as polyhydroxyethylmethacrylate or hydroxyethylmethacrylate, and 50–75% water, and when soaked in a drug solution, they absorb the drug, which is then released slowly in the tear film when the lens is placed on the cornea. Although some studies demonstrated the improved delivery of certain medications via presoaked contact lenses, these devices still represent “pulse‐dose” drug delivery system. An alternative to contact lenses as a drug vehicle is the use of collagen corneal shields, originally developed for use as corneal bandages. The shield is currently fabricated from porcine scleral tissue or bovine dermal collagen. The shield undergoes hydrolysis while in place on the cornea, within 12, 24, 48, or 72 h depending on the degree of collagen cross‐linking determined by titrating the exposure to ultraviolet radiation during the manufacturing process. Ophthalmic inserts are solid or semisolid devices, the size and shape of which are designed for application in the lower fornix. They have been classified as biodegradable (soluble) or nonbiodegradable (insoluble) inserts. The best known nondegradable ocular insert is the Ocusert® Pilo (Alza Corp., USA), a diffusional system designed for controlled release of pilocarpine for seven days. A nonbiodegradable ophthalmic insert containing 5.4 mg phenylephrine and 0.28 mg tropicamide (Mydriasert®, Zeiss‐Meditec, France), which is equivalent to one 10% phenylephrine and 0.5% tropicamide drop, is currently available in Europe to induce mydriasis in human patients prior to cataract surgery. A preliminary evaluation in dogs indicates that mydriasis develops more slowly with Mydriasert than with topical application of the corresponding drugs formulated as eye drops. There are two main approaches for enhancing corneal transfer of topically applied drugs. The first approach is to modify the physicochemical properties of the drug through prodrug derivatization, and the second is to increase transiently the permeability of the corneal epithelium. To overcome the resistance to transport of hydrophilic drugs across the corneal epithelium, a prodrug approach can be considered. Prodrugs are defined as pharmacologically inactive derivatives of drug molecules that are chemically or enzymatically converted to the active parent drugs. An ideal ocular prodrug should be stable and soluble in aqueous solutions to enable formulation, sufficiently lipophilic to pass the corneal barrier, well tolerated, and capable of releasing the active moiety within the eye at a rate corresponding to the therapeutic need. Steroids were perhaps the first class of ophthalmic drugs to which prodrug concept was applied, since the acetate prodrugs of dexamethasone and prednisolone were designed to improve corneal absorption and were found to increase the anti‐inflammatory efficiency by a factor of 1.5–2.0 compared to their parent drugs. Dipivalyl epinephrine (or dipivefrin), developed as an epinephrine prodrug in the late 1970s, is formed by esterification of the hydroxyl groups of the epinephrine molecule. The major advantage of dipivefrin is that a 10‐fold lower dose has a therapeutic effect comparable to that produced by epinephrine, with a significant lowering of systemic side effects and reduction of dose. Topical latanoprost, travoprost, and bimatoprost are all prodrugs of prostaglandin F2α (PGF2α). The periocular routes include the subconjunctival, sub‐Tenon’s, peribulbar, and retrobulbar routes that are used to improve the delivery of drugs to intraocular structures. Their selection depends on the inability of a drug to penetrate the ocular surface, and/or the location of the target site. Among those, subconjunctival injection is the most commonly used in veterinary patients. It can provide high local concentrations for prolonged periods of time, and deliver drugs to both the anterior and posterior structures of the eye. Subconjunctival administration may be indicated for infectious or inflammatory conditions of the cornea and anterior segment because of the expected high and/or sustained drug levels that can be achieved in these ocular structures (Figure 3.5). This route of administration provides therapeutic drug levels for 8–12 h after a single injection of a water‐soluble drug and for up to two to three weeks with drugs in suspension, such as the acetate formulations of corticosteroids. They are used (i) to supplement topical drug delivery; (ii) to replace topical drug when they cannot be used; and (iii) to provide a sustained level of drugs for several days. In a sub‐Tenon’s injection, the Tenon’s capsule is elevated from the sclera with a needle or cannula to place the drug underneath Tenon’s capsule and adjacent to the scleral surface, where little resistance to the diffusion of hydrophilic drugs is thought to occur. Anterior sub‐Tenon’s injections are associated with a higher risk of scleral perforation compared to the subconjunctival injection. Posterior sub‐Tenon’s injection of corticosteroids is indicated in human patients with chronic equatorial and posterior uveitis, and those with cystoid macular edema after cataract surgery or diabetic macular edema. Figure 3.5 Routes of drug distribution after subconjunctival injection. Retrobulbar and peribulbar injections are used to introduce a drug into the orbital cavity. The drug then diffuses rapidly through the orbital tissues and the back of the eye. Several techniques have been proposed for the dog and evaluated in terms of the consistency with which each technique deposits the drug within the retrobulbar cone. Retrobulbar or peribulbar injection is used primarily for regional anesthesia as an adjunctive procedure in ocular surgery to reduce nystagmus and enophthalmos. Potential complications of retrobulbar block include globe perforation, optic nerve injury, extraocular muscle injury, and orbital hemorrhage. Intracameral administration involves delivering a drug directly onto the anterior chamber of the eye. Most commonly, injection or irrigation of the anterior chamber is realized to control iris hemorrhage or pupil size with viscoelastic substances and/or epinephrine during intraocular surgery. Tissue plasminogen activator and carbachol can also be administered intracamerally at the completion of cataract surgery in the dog. Intracameral injection poses a significant risk for local drug toxicity because local intolerance may result in corneal endothelial damage with corneal edema and damage to the iris and lens. The direct administration of a drug solution or suspension into the vitreous cavity is the most efficacious method to provide effective concentrations at the target site and is generally required in threatening posterior segment diseases. However, the potential hazards of the procedure have actually limited its use to the chemical ablation of the ciliary epithelium in end‐stage glaucomatous eyes and less frequently to the treatment of infectious endophthalmitis. The safety of the drug, its vehicle, and its concentration reaching the retina must be taken into account to avoid toxicity. The relatively short half‐life of many intraocularly administered drugs necessitates re‐injections to maintain therapeutic drug concentrations, which predispose to side effects such retinal detachment, vitreous hemorrhage, and bacterial endophthalmitis. As an alternative to multiple injections, delivery systems such as polymeric implants or colloidal carriers have the benefit of providing prolonged activity with controlled drug release within the eye, with a single administration. They can be injected or implanted in the anterior chamber or vitreous cavity, with the advantage of bypassing the ocular permeability barriers. Polymeric implants have been specifically developed for the treatment of human diseases affecting both anterior and posterior segments of the eye, which are not readily accessible by the conventional methods and require prolonged therapeutic drug levels. This approach has been employed in veterinary ophthalmology for the long‐term control of equine recurrent uveitis (ERU) using a sustained‐release system of CSA. Nonbiodegradable devices include reservoir‐type devices, and implant‐type devices such as intravitreal pellets or sclera and punctal plugs, which are able to release drug over a span of weeks or months. In veterinary ophthalmology, systemically administered drugs are used for the treatment of eyelid diseases (i.e., bacterial blepharitis) and orbital infections (i.e., orbital cellulitis or abscess), and can also be used for intraocular conditions, such as bacterial endophthalmitis. However, as discussed previously, diffusion of drugs present in the systemic circulation to anterior segment and posterior segment is curtailed by the BAB and blood–retinal barrier, respectively. Factors affecting the permeability of these barriers include the drug lipophilicity, drug molecular weight, plasma drug levels, and inflammation of the intraocular structures. Ocular iontophoresis, microneedle, and ultrasound‐based ocular drug delivery are noninvasive methods designed to deliver drugs to or thorough the fibrous tunic and intraocular tissues. Antibacterial agents are essential in the successful management of ocular diseases, and used in either a prophylactic or a therapeutic manner. When used prophylactically, selection of antibiotics and factors such as general spectrum of activity, the potential development of resistant organisms, and adverse reactions or toxicities are important. When used therapeutically, the most effective antibiotic agent available is most important. Because of the transcorneal, blood–aqueous, and blood–retinal barriers, the antibacterial agents’ possible routes of administration are also important, and often combined. Lastly, drug‐to‐drug interactions should be considered. When selecting an antibiotic, bactericidal drugs should be used in patients with impaired defenses, as bacteriostatic drugs depend more upon interaction with the natural defenses of the host for maximal efficacy (Box 3.1). When administering a combination of antibiotics, the potential for synergistic or antagonistic effects should be considered. Often antibiotics used commonly topically are not first choice for systemic antibiotics, and hopefully reduce the likelihood of antibiotic resistance. Drugs that inhibit bacterial cell wall synthesis include the penicillins, cephalosporins, bacitracin, and vancomycin. Penicillins may be broadly classified as effective against Gram‐positive bacteria, resistant to penicillinases, exhibiting extended spectra of activity, or effective against Pseudomonas spp. The two primary members of this group are penicillin G (inactivated by gastric acid and thus administered parenterally) and penicillin V (orally administered). Unfortunately, these drugs are highly susceptible to organisms that produce β‐lactamases, including most strains of Staphylococcus aureus and S. epidermidis. Relating to treatment of ocular diseases, the low lipophilicity of penicillin G restricts its passage through the blood–ocular barriers, thereby limiting its efficacy for intraocular infections. Because of the limits of most systemic antibiotics for food animals, the penicillins are used in cattle; administration of penicillin G topically or subconjunctivally, with or without added procaine, achieves therapeutic ocular surface or tear film drug levels, sustained for up to 67 h. The penicillinase‐resistant drugs, including methicillin, oxacillin, cloxacillin, dicloxacillin, and nafcillin, are able to resist bacterial β‐lactamases due to alterations to their chemical structures, thus making them effective against S. aureus and S. epidermidis infections. In cattle, topical administration of benzathine cloxacillin is reportedly effective in the treatment of experimental Moraxella bovis infections. Ampicillin and amoxicillin are the two members of this group, whose spectrum extends beyond Gram‐positive organisms to include Gram‐negative rods. They are inactivated by β‐lactamases; however, addition of sulbactam to ampicillin and clavulanic acid to amoxicillin imparts resistance to β‐lactamases by irreversibly binding the bacterial enzymes, making these two combinations more effective in the treatment of S. aureus and S. epidermidis infections. In horse with bacterial keratitis and ulcerations, 50–100% of Streptococcus equi isolates from horses with bacterial keratitis were susceptible to ampicillin (Table 3.1). In ocular surface of older dogs, increasing resistance to both cloxacillin and amoxicillin–clavulanic acid among Staphylococcus intermedius but not S. aureus isolates has been noted. In cats, orally administered clavulanic acid–amoxicillin was as clinically effective as orally administered doxycycline for the treatment of experimentally induced chlamydial conjunctivitis. Of aerobic bacterial isolates from dogs and cats with orbital disease, 78% and 100%, respectively, were susceptible to amoxicillin–clavulanic acid. Carbenicillin, mezlocillin, piperacillin, and ticarcillin are effective against Pseudomonas aeruginosa and some Proteus and Enterobacter species, Gram‐negative organisms that are resistant to most other penicillins. Ticarcillin has demonstrated in vitro efficacy versus Gram‐positive bacterial isolates from equine eyes with bacterial keratitis, as well as aerobic bacterial isolates from dogs and cats with orbital disease. Cephalosporin antibiotics are composed of a dihydrothiazine and a β‐lactam ring connected to a side chain. The division of cephalosporins into generations is based upon the spectrum of activity conferred by various side chains. Cephalexin, cefadroxil, cefazolin, cephalothin, and cephradine are first‐generation cephalosporins, all with good efficacy versus Gram‐positive organisms and only marginal efficacy versus Gram‐negative organisms. Evaluating isolates from dogs and horses with bacterial keratitis, cephalothin demonstrates good in vitro activity versus β‐hemolytic Streptococcus, with no efficacy versus P. aeruginosa. Cefaclor, cefuroxime, cefoxitin, and cefotetan are second‐generation cephalosporins, which have increased Gram‐negative activity relative to the first generation. Among dogs and cats with orbital disease, 73% and 100% of isolates, respectively, were susceptible to cefoxitin. Ceftiofur, cefotaxime, ceftriaxone, and ceftazidime are third‐generation cephalosporins with increased activity versus enteric Gram‐negative bacteria. Aerobic bacterial isolates from dogs with orbital disease identified 100% susceptibility of isolates to ceftiofur. Cefepime has a broad spectrum versus both Gram‐positive and Gram‐negative organisms, with good demonstrated efficacy versus P. aeruginosa isolates from canine dermatological diseases. Bacitracin inhibits bacterial cell wall synthesis by inhibiting the movement of a peptidoglycan precursor from the cytoplasm through the cell membrane. Its spectrum of action is primarily Gram‐positive, with good activity versus S. intermedius isolates from dogs with bacterial keratitis and β‐hemolytic S. equi isolates from dogs and horses with bacterial keratitis. Bacitracin is a common component of triple antibiotic ophthalmic ointments, as it is unstable in solution. Its combination with neomycin and polymyxin B (both having greater Gram‐negative efficacy) produces a good broad‐spectrum preparation commonly used for prophylaxis (corneal ulcerations) or therapy (nonspecific ocular surface infections). Bacitracin has no appreciable transcorneal penetration in intact corneas, and therefore is of limited value in deep corneal or intraocular infections. The primary side effect of bacitracin that is a potential concern is a local hypersensitivity reaction, Vancomycin inhibits bacterial cell wall synthesis by inhibiting incorporation of the peptidoglycan into the cell wall. It is effective versus Gram‐positive organisms, particularly Staphylococcus spp. (including MRSA) and Streptococcus spp., as well as Clostridium difficile. Due to its spectrum of activity and the development of resistance among organisms highly resistant to other antibiotics, it is imperative to exercise extreme caution in the use of vancomycin in veterinary patients. Ophthalmic use of vancomycin has included topical administration for infectious keratitis and intraocular administration for bacterial endophthalmitis. As bacterial and mammalian cell membranes are quite similar, few antibiotics are able to selectively and effectively target this cellular component without inducing patient toxicity. Polymyxin B and gramicidin are the two most readily used. Polymyxin B disrupts bacterial cell membrane phospholipids, increasing cell permeability and leading to cell death. Its spectrum is limited to Gram‐negative organisms, with good activity versus P. aeruginosa isolates from horses and dogs with bacterial keratitis. Its penetration through an intact corneal epithelium is poor. Gramicidin also alters cell membrane permeability, and its spectrum is directed toward Gram‐positive organisms. Its stability in solution enables its substitution for bacitracin in triple antibiotic ophthalmic preparations. This group of antibiotics includes aminoglycosides, tetracyclines, and macrolides, as well as chloramphenicol. Aminoglycosides inhibit the 30S bacterial ribosome translation of mRNA into protein, and are administered topically or parenterally due to poor oral absorption. In general, the spectrum of activity is strong versus Gram‐negative organisms (P. aeruginosa, Proteus, Escherichia coli, Enterobacter, etc.), while efficacy versus Gram‐positive organisms is restricted to S. aureus. Neomycin, a component of common ophthalmic triple‐antibiotic preparations, is primarily used for prophylaxis in superficial corneal ulceration, or for nonspecific treatment of ocular surface infections. Its primary side effect is contact hypersensitivity. It has limited to no corneal penetration in the presence of an intact corneal epithelium. Consistent with general aminoglycoside susceptibility patterns, S. intermedius isolates from dogs with bacterial keratitis are highly susceptible to neomycin, Pseudomonas isolates demonstrate good susceptibility, but β‐hemolytic Streptococcus isolates are highly resistant. Gentamicin, administered topically or subconjunctivally, is used in the treatment of infectious keratitis, particularly that caused by P. aeruginosa. The commercially available concentration (3 mg/ml) or fortified solutions (13.6 mg/ml) may be utilized in the presence of infection. The ability of gentamicin to provide prophylactic protection is limited due to its Gram‐negative spectrum of activity. In canine corneal epithelial cell cultures, gentamicin in various concentrations adversely affected wound healing. Gentamicin achieves limited transcorneal penetration in normal corneas; however, therapeutic levels are more readily reached in the presence of inflammation. Reports vary significantly regarding development of resistance to gentamicin, indicating no change to increased resistance of P. aeruginosa and S. equi isolates from horses with infectious keratitis (see Table 3.1). Isolates from dogs with bacterial keratitis showed no overall increase in resistance between 1993 and 2003; however, as would be expected, β‐hemolytic Streptococcus strains were more likely to be resistant to gentamicin than were S. intermedius or Pseudomonas strains. Table 3.1 Recommended ophthalmic antibiotic choices based on in vitro susceptibility of organisms isolated from clinical bacterial keratitis cases. Antibiotic (# resistant/total # isolates evaluated, % resistant in reference indicated). a Fluoroquinolones available as ophthalmic preparations were not evaluated. The spectrum of activity and indications for use of tobramycin are similar to those of gentamicin; however, a recent study indicates its efficacy versus Pseudomonas isolates from horses with bacterial keratitis has declined, while Pseudomonas isolates from dogs with bacterial keratitis were uniformly susceptible. As would be expected, efficacy versus Streptococcus isolates is very poor, while efficacy versus S. intermedius isolates is good. Kanamycin has been used to treat infectious bovine keratoconjunctivitis caused by M. bovis. Amikacin is not available as a topical ophthalmic preparation; however, its administration topically has led to therapeutic corneal levels that may be useful in treating patients with ocular infections caused by Gram‐negative bacilli that are resistant to gentamicin or tobramycin. Like the aminoglycosides, tetracyclines interact with the 30S ribosomal subunit to inhibit bacterial mRNA translation. They may be classified as short‐acting (tetracycline, oxytetracycline), intermediate‐acting (demeclocycline), or long‐acting (doxycycline, minocycline). While they have a broad spectrum of bacterial coverage, their clinically relevant spectrum of activity is relatively narrow, due in part to development of efflux mechanisms within bacteria that actively pump drug out of the cells, creating resistant strains. Activity versus rickettsial organisms, Borrelia burgdorferi, Mycoplasma spp., Chlamydophila spp., and Moraxella spp. is consistently strong, while Staphylococcus spp. and Streptococcus spp. may be developing increased resistance. P. aeruginosa is generally considered resistant to tetracyclines, which has been supported by a recent evaluation of isolates from horses with bacterial keratitis. Tetracyclines may be administered topically, orally, or parenterally in patients with ocular disease, with oral administration beneficial for organisms that sequester in nonocular sites (Chlamydophila felis or Mycoplasma spp. in cats, M. bovis
3
Ocular Pharmacology and Therapeutics
Section I: Ocular Drug Delivery
Barriers to Ocular Drug Delivery
Corneal Barriers
Conjunctiva and Sclera Membrane Barriers
Blood–Ocular Barriers
Topical Administration
Ophthalmic Solutions and Suspensions
Drug Disposition After Eye Drop Application
Nasolacrimal Drainage and Tear Washout
Factors Influencing the Drainage Rate
Penetration Across the Cornea
Factors Influencing Corneal Absorption
Penetration via the Conjunctiva/Scleral Route
Systemic Absorption
Mechanisms and Consequences
Systemic Drug Delivery Through Ocular Route
Topical Drug Delivery to the Posterior Eye Segment
Inside the Globe
Drug Distribution
Drug Elimination
Drug Binding
Drug Metabolism
Drug Delivery Kinetics and Ocular Bioavailability
Improvement of Ocular Bioavailability
Improvement of Precorneal Retention
Continuous Infusion of Topical Drugs
Ophthalmic Ointments
Mucoadhesive and Viscosity‐Enhancing Polymers
Colloidal Carriers
Solid Polymeric Devices
Improvement of Corneal Penetration
Ophthalmic Prodrugs
Periocular Administration
Subconjunctival Injection
Sub‐Tenon’s Injection
Retrobulbar and Peribulbar Injections
Intraocular Administration
Intracameral and Intravitreal Injections
Sustained Drug Delivery to Intraocular Tissues
Intraocular Implants
Systemic Administration
Other Methods of Ocular Drug Delivery
Antibacterial, Antifungal, and Antiviral Agents
General Principles of Antibacterial Therapy
Drugs That Inhibit Bacterial Cell Wall Synthesis
Penicillins
Extended‐Spectrum Penicillins
Antipseudomonal Penicillins
Cephalosporins
First‐Generation Cephalosporins
Second‐Generation Cephalosporins
Third‐Generation Cephalosporins
Fourth‐Generation Cephalosporins
Bacitracin
Vancomycin
Drugs That Disrupt the Bacterial Cell Membrane
Gramicidin
Drugs That Affect Bacterial Protein Synthesis
Aminoglycosides
Neomycin
Gentamicin
Canine
Equine
Organism
Tolar et al. (2006)
Ledbetter et al. (2007a)
LoPinto et al. (2015)
Brooks et al. (2000) a
Sauer et al.(2003)a
Keller & Hendrix (2005)
Staphylococcus intermedius
Bacitracin (0/30, 0%)
Chloramphenicol (1/34, 3%)
Ciprofloxacin (0/9, 0%)
Neomycin (2/31, 6%)
Polymyxin (1/31, 3%)
Tobramycin (3/31, 10%)
N/A
N/A
N/A
N/A
N/A
Methicillin‐resistant
Staphylococcus spp.
N/A
N/A
Amikacin (0/17, 0%)
Chloramphenicol (0/17, 0%)
Gentamicin (0/17, 0%)
Neomycin (5/17, 29%)
N/A
N/A
N/A
Streptococcus spp.
Bacitracin (0/19, 0%)
Chloramphenicol (0/19, 0%)
Ciprofloxacin (0/3, 0%)
N/A
N/A
Bacitracin (0/11, 0%)
Chloramphenicol (0/11, 0%)
Bacitracin (0/12, 0%)
Chloramphenicol (0/13, 0%)
Enrofloxacin (2/13, 15%)
Chloramphenicol (0/17, 0%)
Ciprofloxacin (0/5, 0%)
Erythromycin (1/17, 6%)
Gentamicin (3/17, 18%)
Tetracycline (2/17, 12%)
Pseudomonas aeruginosa
Ciprofloxacin (1/15, 7%)
Gentamicin (0/25, 0%)
Neomycin (3/22, 14%)
Polymyxin (0/22, 0%)
Tobramycin (0/22, 0%)
Ciprofloxacin (0/27, 0%) N/A
Ofloxacin (2/27, 7%)
Levofloxacin (0/27, 0%)
Gatifloxacin (1/27, 4%)
Moxifloxacin (3/27, 11%)
N/A
Polymyxin (0/14, 0%)
Neomycin (4/14, 29%)
Enrofloxacin (0/14, 0%)
Ciprofloxacin (0/6, 0%)
Gentamicin (0/6, 0%)
Neomycin (0/3, 0%)
Polymyxin (0/2, 0%)
Tobramycin (0/2, 0%)
Tobramycin
Kanamycin
Amikacin
Tetracyclines
Stay updated, free articles. Join our Telegram channel
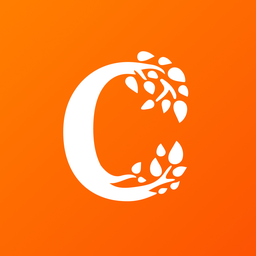
Full access? Get Clinical Tree
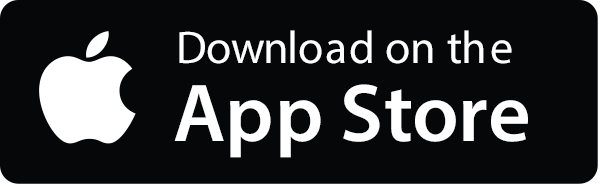
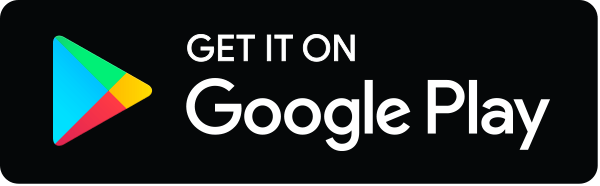