Mark G. Papich Department of Molecular Biomedical Sciences, College of Veterinary Medicine, North Carolina State University, Raleigh, North Carolina, USA Non‐steroidal anti‐inflammatory drugs (NSAIDs) are among the most important drugs used in all species of animals. They have the advantage of producing both analgesic and anti‐inflammatory effects in animals. Because they are not controlled or scheduled drugs, they can be easily prescribed and dispensed. There are a variety of formulations and routes of administration to choose from that include oral tablets, oral caplets, oral liquids, chewable tablets, paste (for horses), oral transmucosal mist, transdermal, and injectable solutions for intravenous (IV) or subcutaneous (SC) administration (Table 24.1, Box 24.1). This chapter will focus primarily on the most common drugs used in most countries, but the reader should appreciate that availability, registration, licensing, and approvals by regulatory authorities vary greatly around the world. There are drugs available in some countries that are not available in others and some older drugs on the market that have been replaced by newer approved animal drugs in some countries. Some older drugs for which new information has not been generated in many years (aspirin for example) will not be covered in this chapter, but details of their pharmacology may be found in previous editions of this and other older textbooks. Although this chapter focuses primarily on the major veterinary species of dogs, cats, horses, and cattle, the use of NSAIDs in other animals should not be overlooked. There has been extensive work in zoo and exotic species, particularly new work published in non‐mammalian species. Surprising differences in both activity (e.g., cyclo‐oxygenase [COX] activity) and pharmacokinetics exist among these other species. But it is beyond the scope of this chapter to address all the other animal species in which NSAIDs are administered; therefore, the reader is encouraged to consult species‐specific publications for more guidance. Information on the pharmacology of these agents has been provided by Lees [1] as well as many other authors [2–5]. Guidelines for clinical use in dogs [2,6–8], cats [7–11], horses [12], and food animals [13] are available in species‐specific reviews. A description of the chemistry, mechanism of action, and clinical use of the coxib class of NSAIDs is also available [14,15]. Because these extensive and complete reviews are available, these details will be abbreviated for this chapter. As shown in Fig. 24.1, arachidonic acid, a fatty acid consisting of 20 carbons, is released from cell membranes by the enzyme phospholipase A2 (PLA2). Arachidonic acid is the predominant fatty acid in animal cell membranes. The products of arachidonic acid biotransformation are referred to as “eicosanoids” because of their structure of 20 carbons (Greek eikosi = 20). Most arachidonic acid is recycled back to the cell membranes, but in the face of various stimuli, arachidonic acid may be acted upon by COX or lipoxygenase (LOX) enzymes to form various eicosanoids, which have a role in homeostasis and disease (Fig. 24.2). Prostanoids are specific eicosanoids that are further metabolized into the various prostaglandins (PGD2, PGF2α, and PGE2), prostacyclin (PGI2), and thromboxane (TXA2). These products act locally via G‐protein‐coupled receptors to generate inflammatory and immunological responses and are also responsible for important physiological functions. A group of LOX products, the leukotrienes, are also responsible for inflammation, but are not inhibited by the traditional drugs covered in this chapter and will not be further discussed. Table 24.1 Classification and pharmacologic parameters for selected non‐steroidal anti‐inflammatory drugs commonly used in animals. Pharmacokinetic parameters derived from references cited in the text. a Apparent volume of distribution (Vd) for most drugs at steady state, unless otherwise noted. b Percent bioavailability (F%) after oral administration. Colored portions of the structural diagrams represent the reactive groups. The action of traditional NSAIDs is to inhibit COX enzymes and alter the formation of the prostanoids [16]. The significant development that improved the understanding of NSAIDs’ mechanism of action occurred in the early 1990s when new details about the targets of these drugs emerged [17,18]. It was elucidated that there are two isoenzymes (isoforms) of COX (also known as “prostaglandin synthase”) that are responsible for synthesis of prostaglandins. Prostaglandin synthase‐1 (COX‐1) is usually simplistically described as a constitutive enzyme expressed in tissues [17,18]. Prostaglandins, prostacyclin, and thromboxane synthesized by this enzyme are responsible for normal physiologic functions. Prostaglandin synthase‐2 (COX‐2), on the other hand, is inducible (upregulated) and synthesized by macrophages and inflammatory cells after stimulation by cytokines and other mediators of inflammation. In 2002, a third isoenzyme of COX was described [19,20]. The COX‐3 enzyme is a splice variant from the COX‐1 gene, which to date has only been identified in the canine brain, human brain, and heart [20]. It is hypothesized that the mechanism of action of acetaminophen (paracetamol) is by inhibition of COX‐3 although this hypothesis predated the discovery of the COX‐3 splicing variant [19,20]. Acetaminophen may have other mechanisms of action, as discussed later in this chapter. Figure 24.1 Membrane phospholipids are the source of arachidonic acid, the major eicosanoid in animals. Arachidonic acid is further metabolized by COX enzymes to the various prostaglandins (PGs), prostacyclin, and thromboxane to produce clinical, immunologic, and physiologic functions. The COX enzymes are the targets for non‐steroidal anti‐inflammatory drugs. Figure 24.2 Metabolism of arachidonic acid products. The cell membrane generates arachidonic acid via de‐esterification by the enzyme phospholipase A2. Arachidonic acid is further metabolized by cyclo‐oxygenase enzymes (COX‐1 and COX‐2) to the various prostaglandins (PGs), prostacyclin (PGI2), and thromboxane (TXA2) to produce clinical, immunologic, and physiologic functions. An alternative pathway is via the lipoxygenase enzyme (LOX), which produces the inflammatory leukotrienes (LTs). The COX enzymes are the targets for non‐steroidal anti‐inflammatory drugs, but do not ordinarily affect the LOX enzymes. An exception to this traditional view of COX enzyme inhibition by NSAIDs is the action of grapiprant, a new class of drugs that can technically be considered “non‐steroidal anti‐inflammatory drugs,” but do not inhibit the COX enzyme. This agent will be discussed in more detail later in the chapter. Instead of inhibiting production of prostaglandins, it blocks the prostaglandin receptor. After inflammation is triggered and prostaglandins are synthesized, prostaglandin‐E2 (PGE2) exerts its effects via four receptors (EP1‐4). The EP4 receptor is responsible for some of the sensitization of sensory neurons and signs of inflammation. Although other receptors are activated during inflammation, the EP4 receptor has been identified as the primary receptor responsible for mediating pain and inflammation associated with osteoarthritis. One of the new “non‐traditional” NSAIDs, also called “piprants,” is grapiprant, which blocks this receptor. The explanation of COX function described above is likely too simplistic. Both enzymes can participate in regulatory and homeostatic function, and both can produce pain and inflammation. The predominant enzyme reflects the tissue or cell type in which it is produced. For example, COX‐1 is the dominant enzyme in platelets, and COX‐2 is the dominant enzyme in inflammatory cells. In bone, COX‐1 is expressed under normal conditions, but COX‐2 is expressed during mechanical stress. Some COX‐2 products are important for homeostatic function, and COX‐1 products are involved in pain and inflammation. Prostaglandin synthesis is inherently higher in canine gastric mucosa than duodenal mucosa [21,22], but COX‐2 production of prostaglandins can be upregulated in these tissues, responding to inflammatory stimuli to produce a protective and healing role [23]. COX‐2 products are also important in normal renal function. COX‐2 may be upregulated under conditions that may be stressful to the kidney, such as hypovolemia or severe hypotension [24,25]. Therefore, the perception that COX‐2 is a “bad” enzyme and COX‐1 is a “good” enzyme is too simplistic because we now understand that there is some overlap in the functions of these isoforms on organs [26]. Drugs that inhibit these enzymes (e.g., NSAIDs) can be effective and/or harmful regardless of whether they are selective or non‐selective in their action on these enzymes. Nevertheless, some of the most recently developed NSAIDs have targeted COX‐2 inhibition, so that COX‐1 is spared as much as possible with the goal of producing analgesia and suppressing inflammation without inhibiting physiologically important prostanoids, particularly the COX‐1 products in the gastrointestinal tract. Although it is assumed that prostaglandin inhibition in both peripheral and nervous system (spinal and brain) tissues is the most important mechanism of action for most NSAIDs, there may be other mechanisms to explain some of their effects. These properties have not been accepted as a mechanism compared to prostaglandin inhibition but deserve some consideration. Some NSAIDs, including salicylates, may inhibit nuclear factor kappa‐B (NF‐κB), which promotes synthesis of other inflammatory mediators. There is not universal agreement on the mechanism of action of carprofen, which is the most commonly prescribed NSAID in dogs. Carprofen significantly inhibited PGE2 (a biomarker for COX‐2) directly at sites of inflammation in dogs, providing evidence that carprofen does indeed act on COX‐2 [27]. Other evidence indicates that it inhibits prostaglandins in vitro [28], but carprofen did not show an in vivo antiprostaglandin effect in dogs [29,30]. In another study, the investigators were unable to show that carprofen inhibited either COX‐1 or COX‐2 [31], suggesting either a central mechanism of action or activity on other pathways. In horses, similar questions have been raised [32] with a conclusion that the anti‐inflammatory effects of carprofen in horses were not caused by COX inhibition. In another study in the same laboratory [33], they concluded that recommended doses of carprofen produce minimal inhibition of COX, and it is likely to achieve its therapeutic effects at least partially through other pathways, possibly including weak to moderate inhibition of 5‐lipoxygenase and enzyme release. Other investigators [34] also concluded that there may be COX‐independent mechanisms for some NSAIDs in the equine intestine. Selectivity of COX‐2 versus COX‐1 has been expressed as the COX‐1:COX‐2 inhibitory ratio. This ratio is derived from studies in which the inhibitory effect, usually expressed as the inhibitory concentration to inhibit 50% of activity (IC50), is measured from stimulating cells that are capable of expressing products of these enzymes. In the whole blood assay, the source for COX‐1 products (thromboxane or TXA2) is platelets and the source of COX‐2 products (PGE2) is leukocytes. The ratio is expressed as COX‐1 [IC50]:COX‐2 [IC50], or simply COX‐1:COX‐2. The higher the value above 1.0, the greater the inhibitory action of the drug is for COX‐2 compared to COX‐1. There is subjective value placed on the magnitude of the ratio when considering the drug as “COX‐1 sparing,” “COX‐2 specific,” “COX‐2 preferential,” or “COX‐2 selective.” These terms have been used by other investigators [35], but the magnitude of the ratios that define these criteria has not been agreed upon. In reality, COX‐2 selectivity is a continuous variable that does not easily allow a distinct definition of COX‐2 selective or non‐selective. These are relative definitions, rather than absolute categories of NSAIDs. The drugs with the highest inhibitory ratios are the selective COX‐2 inhibitors (based on in vitro assays for the COX‐2 enzyme) often called the “coxibs” or “coxib class of NSAIDs.” These drugs include the veterinary products firocoxib, deracoxib, robenacoxib, and mavacoxib and the human product celecoxib (other coxibs such as rofecoxib have been withdrawn from the human market because of adverse effects on the cardiovascular system in human patients). Carprofen and meloxicam can be considered somewhat COX‐2 preferential. Ketoprofen is non‐selective, and etodolac has been classified as somewhat preferential to non‐selective, depending on the study referenced. Aspirin is highly COX‐1 selective. Although the IC50 ratio is the normal convention used for determination of COX selectivity [4,5], some investigators have proposed that a higher inhibitory concentration ratio (e.g., IC80) may be better for predicting clinical efficacy, because such a high degree of inhibition may be necessary for analgesia or anti‐inflammatory effects [36]. Comparisons of COX‐1 versus COX‐2 inhibition of veterinary NSAIDs vary among the drugs, animal species, and study technique used [15,37]. For example, deracoxib is considered a highly selective COX‐2 inhibitor based on an assay performed in purified enzymes [38]. In this study, the COX‐1:COX‐2 ratio was 1275, much higher than other drugs tested. But when tested using canine whole blood and compared to other NSAIDs, deracoxib had a ratio of only 12 [39]. In the same study, carprofen had a ratio of 6–7 and firocoxib had a ratio of 384–427 [39]. Although etodolac is no longer used in dogs, there was a tremendous difference between the canine and human COX‐1:COX‐2 ratio, with a value of 8.1 in humans but 0.52–0.53 in dogs [40]. In a study using canine enzyme systems, carprofen had a COX‐1:COX‐2 ratio of 129 [28], but in another study, using cell lines of another species (sheep and rodent), the ratio was 1.0 [16], and in a study using canine macrophages, the ratio was 1.75 [41]. Yet another study on carprofen showed a ratio of 5.3, which was 1000 times less potent in whole blood than in cell culture [42]. Large differences among animal species have been shown for carprofen [5,33], which may be attributed to effects of carprofen on human cells being relatively selective for COX‐1, but for canine and feline cells, carprofen shows preferential activity against COX‐2, and for equine cells, it is non‐selective or slightly preferential for COX‐2. In view of the discrepancies among studies and techniques, it is generally accepted that the whole blood assay should be the gold standard for determining COX‐1/COX‐2 specificity because it most closely simulates the appropriate physiologic conditions [4,5,35,36,43]. The advantage of the whole blood assay is that it incorporates the components into the assay that normally occur in circulating blood, namely proteins, cells, platelets, and enzymes. These components are not present in isolated cells or enzyme systems used for some earlier assays. Because the NSAIDs are highly protein bound (as discussed below), this is particularly important as only a small fraction, the unbound fraction, is biologically active in blood. The whole blood assay measures COX‐2 products (PGE2) from stimulated leukocytes and COX‐1 products (TXA2) from stimulated platelets. Pharmacokinetic parameter estimates for NSAIDs are available from a variety of research reports and the drug package inserts from sponsors. Some selected values are shown in Table 24.1 and are derived from a variety of sources. It is not possible to provide an exhaustive list of every study because there are hundreds of pharmacokinetic studies published for NSAIDs in animals. The common features possessed by the NSAIDs used in animals are worth noting. All have high protein binding (> 90%). Generally, all are well absorbed after oral administration and are weak acids that have high lipophilicity at physiologic tissue pH. The lipid/water partition coefficient, reported as the logarithm of the partition coefficient, or LogP, for these drugs is greater than or near 2.0. If the LogP for a medication is close to or above 2.0, diffusion across biologic membranes is favored (unless restricted by protein binding) and oral absorption is favored by high lipophilicity. Being weak acids, the lipophilicity (LogD) is highest at the low pH of the stomach and is lower, but still above 0.0, at the physiologic pH of 7.0. Meloxicam is an exception because the LogD is –0.75 at a pH of 7.0. Mavacoxib is another exception because it is a weak base. Both lipophilicity and protein binding affect a drug’s volume of distribution. The volume of distribution varies considerably among the NSAIDs (Table 24.1). For many drugs, high protein binding is associated with a small volume of distribution because protein binding decreases diffusion out of the extracellular compartment (e.g., plasma and extracellular fluid), but this is not a consistent feature of the NSAIDs. Carprofen and phenylbutazone have the lowest volume of distribution at 0.14 L/kg (lower than extracellular water volume), and most NSAIDs have volumes of distribution less than 1.0 L/kg. However, firocoxib, deracoxib, and etodolac all have volumes of distribution greater than 1.0 L/kg. Firocoxib has the highest volume of distribution (approximately 4.6 L/kg in dogs) [44], while it has the lowest lipophilicity (LogP 1.96). Interestingly, carprofen has one of the highest lipophilicity values among the NSAIDS (LogP of 3.79), but also has the lowest volume of distribution. The estimated plasma half‐life varies tremendously among the NSAIDs, and among species (Table 24.1). For example, in dogs, the half‐life is < 1 h for robenacoxib, 1.6 h for ketoprofen, 3 h for deracoxib, and up to 24 h for meloxicam. Phenylbutazone ranges from 5–6 h in dogs and horses to over 50 h in cattle. Firocoxib has a half‐life of 7.8 h in dogs, but 30 h or more in horses. Mavacoxib, an NSAID approved for dogs in Europe but not the United States (US), is unusual in that it has very low systemic clearance, resulting in a half‐life in laboratory Beagles of 15.5–19.3 days [45]. However, in osteoarthritic dogs of other breeds, the half‐life was highly variable with an average of 44 days but exceeding 80 days in some dogs [46]. Despite these differences, the half‐life does not appear to influence dosing intervals. In other words, the canine‐approved labels for these drugs generally state once‐daily oral administration for osteoarthritis or soft tissue inflammation despite the disparate half‐lives. Mavacoxib is a notable exception with initial dosing at a 14‐day interval with subsequent doses given every 30 days [46]. One of the reasons why plasma drug pharmacokinetic parameters (e.g., half‐life) may not predict the dose interval is because of a lag time between the tissue concentrations and blood concentrations, raising questions about the value of plasma or serum pharmacokinetics to predict dose and dose intervals. Even the drugs with short half‐lives can be administered once daily because the tissue concentrations and inhibitory effects persist much longer. Protein binding has historically been used to explain the long persistence in tissues, but these drugs are bound in plasma as well as tissues, which challenges this assumption. The high lipophilicity of these drugs also may explain the long tissue half‐lives because high lipophilicity favors intracellular distribution, which can serve as a reservoir for prolonged tissue levels. The concept of “ion trapping” has been used to explain the high concentrations of NSAIDs in tissues compared to plasma. However, this is a false application of this principle. NSAIDs generally have a low pKa (weak acids); therefore, they are ionized in both plasma and tissue. The pH in tissues, even when inflamed, is generally not below the pKa, making ion trapping unlikely because of the small differential between tissue and plasma pH, compared to the pKa of many NSAIDs, which have a pKa in the range of 4–5. In other words, the tissue pH does not fall below the pKa of these NSAIDs for ion trapping to factor into the drug’s distribution. The NSAIDs are biotransformed by hepatic mechanisms, which is characteristic of lipophilic, poorly water‐soluble compounds that have relatively low systemic clearance. Clearance values are not listed in Table 24.1, but all NSAIDs have clearance values of approximately 0.4 L/kg/h (6.67 mL/kg/min) or less. With liver blood flow being approximately 30 mL/kg/min in dogs [47], such a low value for these NSAIDs would qualify them as low clearance compounds. Mavacoxib is an extreme example with systemic clearance of only 0.0027 L/kg/h in Beagle dogs [45]. Similar properties are observed for the NSAIDs in other species in which the systemic clearance is much lower than liver blood flow. There are a few exceptions: systemic clearance of meloxicam in horses is approximately three to five times higher than in humans and three times higher than in dogs [48,49]. Robenacoxib has a clearance of approximately 9–12 mL/kg/min in cats [50,51] and 13 mL/kg/min in dogs [51,52], which is higher than the other NSAIDs, and it also has a correspondingly short half‐life in each of these species. Gastrointestinal absorption is generally high for the formulations approved for animals (above 90% and often close to 100%), but there are exceptions. Firocoxib and mavacoxib are unusual with only 38% and 46% oral bioavailability in dogs, respectively. Robenacoxib has unusually low bioavailability in cats that is significantly affected by feeding [51]. There are also lower values for oral absorption of some drugs in horses and cattle (Table 24.1). Feeding may increase, inhibit, or delay absorption of concurrently administered NSAIDs. For example, feeding horses at the same time as oral phenylbutazone administration does not decrease absorption, but it is delayed significantly, presumably because of binding to materials in feed and release in the distal intestine [53]. Robenacoxib is highly affected by feeding with oral absorption of 10% in fed cats versus 49% in fasted cats [51,54] and 62% in fed dogs versus 84% in fasted dogs [51,52]. Mavacoxib (unique because it is a weak base instead of a weak acid) is affected in the opposite direction, with feeding increasing the oral absorption from 46% to 87% [45]. Meloxicam has good oral absorption and is only slightly affected by feeding in horses (Table 24.1). The propionic acid derivatives (carprofen and ketoprofen) exist as R(–) and S(+) chiral enantiomers. Chirality is important because each isomer may exhibit different pharmacokinetic and pharmacodynamic behavior. For example, the S(+)‐enantiomer (eutomer) is most active (lowest IC50) for prostaglandin inhibition for these two NSAIDs. Etodolac also exists as a chiral enantiomer, with the S(+)‐isomer being more active. None of the other drugs listed in Table 24.1 have chiral enantiomers. The association between the drug’s pharmacokinetics and action at the target site has been the focus of pharmacokinetic‐pharmacodynamic (PK‐PD) studies. If the plasma or serum pharmacokinetic properties can be compared to the pharmacodynamic studies that measure COX‐1 and COX‐2 inhibitory concentrations, or observations of clinical response, it allows investigators to use the estimated pharmacokinetic parameters of the drug to derive optimal and safe dosing for animals. These approaches have been used to evaluate various NSAIDs used in animals [4,5,55–57]. For example, this approach was used to characterize the dose for robenacoxib in dogs and cats [50,51,55–59] and was used to evaluate NSAIDs for horses [48,49]. This approach entails the use of a blood concentration corresponding to 80% inhibition of COX‐2 (IC80) to produce a therapeutic effect, and a 20% inhibition of COX‐1 (IC20) to avoid adverse effects. A value of 80% COX‐2 inhibition is used to predict clinical effects because a drug concentration that inhibits the enzyme by only 50% may not be enough to produce a therapeutic effect. Using these inhibitory concentration values as targets, PK‐PD modeling can be used to derive doses to attain optimal blood concentrations [36,60]. The doses derived are intended to be starting points from which other studies investigating clinical safety and effectiveness can be planned. For example, using the PK‐PD modeling approach, investigators determined a dose of meloxicam of 0.17 mg/kg every 24 h in cats [56]. This dose of meloxicam deviates from doses derived from clinical studies because it is below the US Food and Drug Administration (FDA)‐registered single dose (0.3 mg/kg) for cats [61] but higher than the chronic dose used clinically and registered in other countries, including Europe [62]. On the other hand, this approach correctly identified the optimum dose of 2 mg/kg of robenacoxib in cats [50,51,57,59,63] and dogs [51,64]. Rather than using an in vitro assay such as the whole blood assay to measure COX inhibition as the pharmacodynamic surrogate marker for efficacy, another approach is to use an in vivo measure. An in vivo measure is more likely to reflect physiologic and pathologic conditions and predict clinical outcome. Several in vivo models have been used to test NSAIDs in animals, which are described in other reports [4,27]. A typical in vivo model involves inducing inflammation in a tissue cage and measuring the inhibition of the inflammatory response in relation to the drug concentration. Alternatively, the model may use an injection of an irritant into a joint followed by an observation of the response by measuring the degree of lameness produced, heat, and/or pain [39,49,50]. Values such as 50% inhibitory concentration (IC50) can be estimated from the in vivo model as it was for the in vitro model and used to derive an effective dose. One example of this approach was used to derive a dose for meloxicam in cats. Inflammation was induced in experimental cats, and the response was matched to pharmacokinetic parameters for PK‐PD modeling [55]. These investigators calculated a single dose of 0.25–0.3 mg/kg of meloxicam to produce optimum analgesic, anti‐inflammatory, and antipyretic effects. This dose agrees with the dose derived from clinical trials that led to the current FDA‐registered dose for cats in the US [61], but is higher than the dose used clinically by many practitioners. In a tissue study of carprofen in dogs [27], the investigators showed that carprofen persists in tissue sites much longer than in plasma. But, contrary to popular views, the concentration in the inflamed tissue was not different from healthy tissue. Carprofen significantly decreased PGE2 in the inflamed tissue. The mechanisms of action described above do not apply to other agents used for acute and chronic pain in animals. Grapiprant is technically classified as an NSAID, but it does not inhibit the COX enzyme. Acetaminophen is often not listed with other NSAIDs because it does not have anti‐inflammatory properties and the mechanism of action has yet to be fully identified. However, acetaminophen will be listed in this chapter because it is sometimes considered as an oral treatment option for dogs, and more recently for horses. Dipyrone (metamizole in some countries) is also listed in this chapter with the NSAIDs. It lacks significant anti‐inflammatory effects, and the mechanism of action is not entirely understood. However, it has some COX inhibiting activity, is used in small animals for acute pain in some countries, and is approved for use in horses in the US. Grapiprant is a non‐COX‐inhibiting agent. Instead of inhibiting production of prostaglandins, it blocks the prostaglandin receptor. After inflammation is triggered and prostaglandins are synthesized, PGE2 exerts its effects via four receptors (EP1‐4). The EP4 receptor is responsible for some of the sensitization of sensory neurons and signs of inflammation. Although other receptors are activated during inflammation, the EP4 receptor has been identified as the primary receptor responsible for mediating pain and inflammation associated with osteoarthritis. In dogs, grapiprant has high binding affinity for the EP4 receptor and binds competitively at a single site [65,66]. The effective dose in dogs, based on PK‐PD analysis, and extrapolation from laboratory animal models of pain, is 1.7 mg/kg [65], which is almost the same as the FDA‐approved dose of 2 mg/kg once daily. In a pivotal clinical study [67], grapiprant showed effectiveness comparable to traditional COX inhibiting drugs in dogs with naturally occurring osteoarthritis. In dogs with osteoarthritis, 48% were classified as treatment successes compared to 31.3% of placebo‐treated dogs (131 dogs in each group). In studies in which grapiprant has been compared to other NSAIDs for treatment of acute pain, it did not perform as well. Compared to firocoxib in a model of acute pain [68], it was not as effective, and in dogs with experimentally induced synovitis, grapiprant was the least effective agent, compared to carprofen and another experimental drug [69]. In another study performed in dogs undergoing ovariohysterectomy surgery, there was no difference between carprofen and grapiprant using a validated scoring system for postsurgical pain [70]. However, these were acute pain models and may not indicate effects during chronic pain treatment. Adverse reactions in dogs have been minimal and are typical as for traditional NSAIDs. These may include vomiting, diarrhea, and decreased appetite, which can be observed after administration of other NSAIDs. These events have been mild and usually transient. In a safety study with research dogs, they tolerated a dose equivalent to 30 mg/kg of the tablet for 9 months with adverse effects attributed to the gastrointestinal tract characterized as mild and infrequent [71]. The pharmacokinetics in cats were similar to dogs [72]. In a safety assessment for cats [73], it was safe at doses suggested for therapeutic use (similar dose as for dogs). However, clinical efficacy in cats has not been established. Grapiprant has not been evaluated in horses, except for pharmacokinetic studies [74]. The plasma concentrations have been low, and it was concluded that it will likely require a higher dose than the canine dose of 2 mg/kg to achieve efficacy. Acetaminophen has been administered alone or in combination with opiates (codeine, oxycodone, or hydrocodone). Acetaminophen should never be prescribed for cats because of the potential for severe toxicity [75]. However, it is safe in dogs, even when administered at high doses [75]. Acetaminophen has been considered when oral treatment is needed for dogs that are refractory to traditional NSAIDs alone or have adverse effects from NSAIDs. Acetaminophen has not produced renal or gastric injury in dogs when prescribed at 15 mg/kg orally, every 8–12 h. Evidence of toxicity was not observed in dogs until doses of 100 mg/kg were exceeded [75]. Although it produces mild analgesic effects, acetaminophen does not produce anti‐inflammatory effects at clinically relevant doses [76,77]. A study in a canine surgery model that demonstrated anti‐inflammatory effects used doses that are higher than recommended clinically [76]. On the other hand, when acetaminophen was administered at a dose of 15 mg/kg every 8 h to dogs, there were no differences between treatments when acetaminophen was compared to carprofen or meloxicam for analgesia in dogs after ovariohysterectomy surgery [78]. In experimental dogs with joint pain, acetaminophen combined with codeine was not as effective as oral carprofen [79]. There are no studies on the long‐term use for osteoarthritis in dogs. In people, acetaminophen has not been effective (or minimally effective) for the treatment of osteoarthritis of the hip and knee [80]. Most of the experience with clinical use of acetaminophen in dogs has been anecdotal as an alternative to NSAIDs or opioids. It has been administered to dogs in combination with codeine, oxycodone, and hydrocodone, despite a lack of clinical studies on the effectiveness of these preparations. Codeine absorption is low in dogs, and there are no studies that demonstrate that it produces convincing analgesia. The mechanism of acetaminophen’s action is unknown, but there are a few proposed theories [81,82]. Although it has long been assumed that acetaminophen does not inhibit the COX enzyme, it may be a COX inhibitor but act in cells in which low concentrations of arachidonic acid are present [81]. The site of acetaminophen action may be the peroxidase enzyme component of prostaglandin‐H2 synthase [83]. Prostaglandin‐H2 synthase consists of both peroxidase and COX portions but is collectively referred to as “COX” in most papers. The target for traditional NSAIDs is the COX portion of prostaglandin‐H2 synthase. COX inhibition probably occurs at site‐specific tissues, sparing the gastrointestinal mucosa, platelets, and kidneys, but acting centrally [20]. It may also be a selective COX‐2 inhibitor in selected tissues [84]. Other evidence presented to support the analgesic efficacy of acetaminophen is that it appears to inhibit the COX‐1 variant referred to as “COX‐3” [19]. This action may be more prominent in dogs than in any other species. COX‐3 effects occur in the brain without affecting prostaglandin synthesis at other sites in the body (e.g., kidney and gastrointestinal mucosa) that could potentially lead to adverse effects. Other non‐traditional NSAIDs (dipyrone and phenacetin) also appear to inhibit COX‐3. Because COX‐3 may be responsible for pyrexia and mediating central pain, this may explain the efficacy of acetaminophen for the treatment of mild pain and fever reduction. On the other hand, in other review papers, the authors have not supported this mechanism for the action of acetaminophen [83,85,86], and other mechanisms discussed in this section may be more important.
24
Non‐Steroidal Anti‐Inflammatory Drugs
Introduction
Mechanism of action
Metabolism of arachidonic acid
Drug
Chemical group and structure
COX‐1 or COX‐2 inhibitory
Half‐life (t1/2)
Apparent volume of distribution (Vd) a
Bioavailability (F%) b
Protein binding
Acetaminophen (Paracetamol)
Aminophenol
No significant COX activity
Dogs: 1–4 h (depending on the study)
Cats: 0.6 h
Horses: 2–5 h (depending on the study)
Dogs: 0.87–1.3 L/kg
Dogs: 44–100% (depending on the study)
Carprofen
Propionic acid
Preferential for COX‐2
S(+)‐enantiomer
Dogs: 11.7 h (100 mg IV bolus) and ~ 8 h (range 4.5–9.8 h, oral)
Horses: 8–20 h (depending on the study)
Cats: 20±16.6 h (range 9–49 h); and 10.7±5.17 h
Calves: 37±2.4 h
Cows: 30.7±2.3 h (43±2.3 h with mastitis)
Dogs: 0.14±0.02 L/kg
Cats: 0.14±0.05 L/kg; and 0.25 L/kg
Horses: 0.307±0.036 L/kg
Calves: 0.163±0.002 L/kg
Cows: 0.091±0.003 L/kg
Dogs: > 90% (oral)
Dogs: > 99%
Deracoxib
Coxib (diaryl substituted pyrazole)
Selective for COX‐2
Dogs: 3 h (2–3 mg/kg IV)
Cats: 7.9 h
Dogs: ~ 1.5 L/kg
Dogs: > 90% (2 mg/kg, oral)
Dogs: > 90%
Robenacoxib
Coxib
Selective for COX‐2
Dogs: 0.63 and 1.1 h
Cats: 1.49, 1.87, 0.84, and 0.78 h (depending on the study)
Dogs: 0.24 L/kg
Cats: 0.13 and 0.19 L/kg (depending on the study)
Dogs: 84% (non‐fed); 62% (fed)
Cats: 49% (non‐fed); 10% (fed)
Dogs: 98%
Cats: 99.9%
Etodolac
Pyranocarboxylic acid
Non‐selective for COX‐2 or preferential for COX‐2 (depending on the study)
Dogs: 7.66±2.05 h (oral, fasted); 11.98±5.52 h (oral, non‐fasted); 9.7±0.97 h (IV)
Horses: 2.85 h (mean)
Dogs: 1.14 L/kg
Horses: 0.29±0.09 L/kg
Dogs: ~ 100%
Horses: 77% (range 43–100%)
Dogs: > 95%
Firocoxib
Coxib
Selective for COX‐2 (most highly selective of all the veterinary NSAIDs)
Dogs: 7.8 h
Horses: ~ 30–40 h
Dogs: ~ 4.6 L/kg
Horses: 1.7 L/kg
Dogs: ~ 38%
Horses: 80–100%
Dogs: 96%
Horses: > 97%
Grapiprant
Piprant
No COX activity; antagonist of the prostaglandin‐E2 (PGE2) receptor 4 (EP4)
Dogs: 1.95–5.42 h
Cats: 4.4 h
Horses: 5.86 h
Dogs: 1.9 L/kg
Cats: 0.92 L/kg
Dogs: 90% (higher in fasted)
Cats: 40%
Dogs: 95%
Ketoprofen
Propionic acid
Non‐selective for COX‐2
S(+)‐enantiomer
Dogs: 1.65 ±0.48 h (oral)
Cats: 1.5 h
Horses: 1–7 h (depending on the study)
Calves: 0.42–2.19 h (depending on study and age)
S(+)‐enantiomer
Dogs: 0.39±0.07 L/kg
Horses: 0.14±0.02 L/kg and 0.22±0.08 L/kg
Calves: 0.26±0.06 L/kg
Dogs, Cats: almost 100%
Horses: 70–100%
Horses: 93%
Mavacoxib
Coxib
COX‐2 selective
Dogs: 17 days median (range 8–39 days in Beagle dogs) and 39 days (in field trial dogs, with some dogs > 80 days)
Dogs: 1.6 L/kg
Dogs: 46.1% (fasted), 87.4% (fed); dose based on fed dogs
Dogs: 98%
Meloxicam
Enolic acid derivative (oxicam)
COX‐2 preferential, but at high doses COX‐2 specificity diminishes
Dogs: 24.0±26.2% h (IV), 23.7±30.0% h (oral)
Cats: 26–37 h (depending on study)
Horses: 5–12 h (depending on the study and formulation)
Cattle: 20–30 h (depending on the study), 14.6 h (cows)
Dogs: ~ 0.32±20% L/kg (Vd‐area)
Horses: 0.23 and 0.12 L/kg (depending on study)
Cats: 0.245 L/kg (Vd/F)
Cattle: 0.194 L/kg
Dogs: 100%
Horses: 75–100%
Cattle: 100%
Dogs: 97%
Horses: 98.5%
Phenylbutazone
Pyrazolone (pyrazolidinedione)
Non‐selective for COX‐2
Horses: 5 and up to 7 h (depending on the study)
Calves: 53.4 h
Bulls: 62 h
Cows: 36–55 h (depending on the study)
Dogs: 4–6 h
Cattle: 0.13–0.14 L/kg
Horses: 0.14 L/kg
Cattle: 54–69% and 69–89% (depending on the study)
Horses: 70% (delayed with feeding)
Horses: 99%
Cattle: 93–98%
Flunixin
Anthranilic acid (pyridinecarboxylic acid)
Non‐selective for COX‐2
Horses: 2–3 h (in most studies)
Cows: 3–8 h (depending on the study)
Dogs: 3.7 h
Cats: 6.6 h
Horses: 0.15±0.04 L/kg
Dogs: 0.18±0.08 L/kg
Cats: 0.75 L/kg
Cows: 0.42, 0.78, and 0.50 L/kg in different studies
Dogs: ~ 100%
Horses: 72%
> 90% in all animals studied
Dipyrone (Metamizole)
Pyrazolone
Mechanism uncertain; may inhibit COX‐3 or act through other mechanisms
All values based on the major active metabolite.
Horses: 3–6 h
Dogs: 5–6 h
Cats: 6–7 h
All values based on the major active metabolite.
Horses: 1.4–1.6 L/kg
Dogs: 5 L/kg
Cats: 0.967 L/kg
All values based on the major active metabolite.
Dogs: 100%
Cats: 81%
Not reported
Other possible mechanisms of action
COX‐1 versus COX‐2 inhibition
Pharmacokinetic properties
Stereoisomerism
Pharmacokinetic‐pharmacodynamic properties
In vivo assessment of PK‐PD models
Unique features of non‐traditional NSAIDs
Grapiprant
Acetaminophen (Paracetamol)
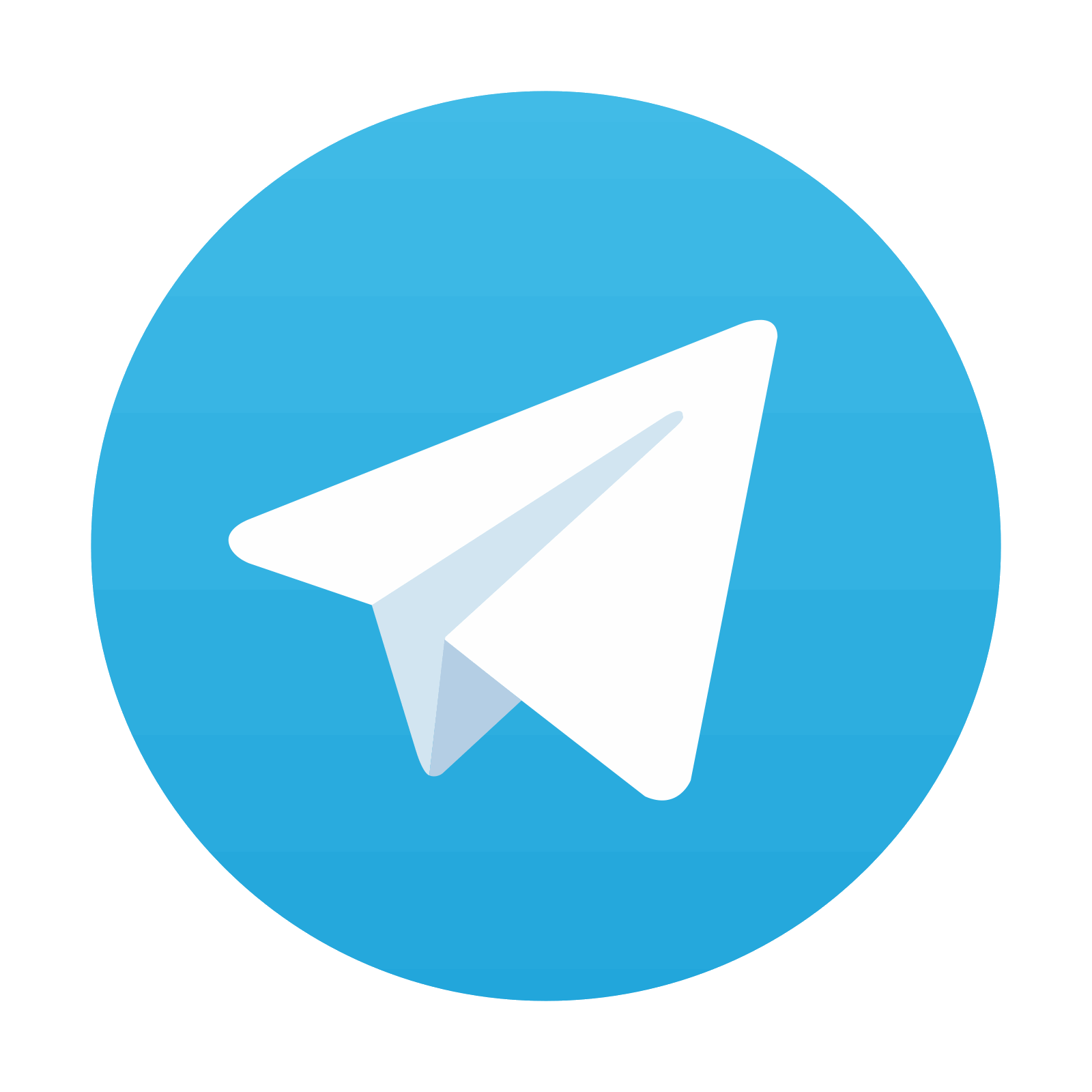
Stay updated, free articles. Join our Telegram channel
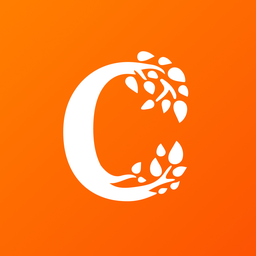
Full access? Get Clinical Tree
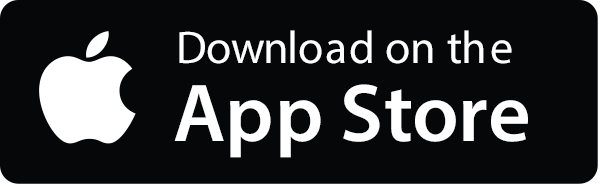
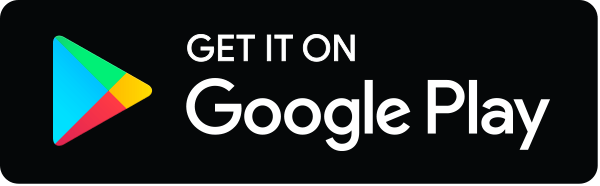