Manuel Martin‐Flores Department of Clinical Sciences, College of Veterinary Medicine, Cornell University, Ithaca, New York, USA Long before its introduction to medical practice, curare was extracted primarily from plants, such as Chondrodendron species, by native South American aboriginal populations for use in quivers, arrows, or spears to hunt animals. Curare’s site of action, the neuromuscular junction (NMJ), was described by Claude Bernard as early as 1844 through his well‐known experiments using frog nerve–muscle preparations, demonstrating that direct muscle stimulation could be achieved while evoked contractions were abolished when motor nerves were stimulated in the presence of curare. This discovery was preceded by observations by Benjamin Brodie in 1812 who described that animals injected with curare could be kept alive if their lungs were ventilated. Later, in 1934, Henry Dale described the role of acetylcholine (ACh) in neuromuscular transmission. A multitude of studies that followed those original observations described the anatomy and function of the NMJ and the neurotransmitters involved. The NMJ and the events that result in neuromuscular transmission are now well understood and an immense amount of information has been made available since those initial reports. Curare was first introduced to medical practice as a potential treatment for convulsive seizures and spasticity and as part of shock therapy. It was then recognized that the effects of curare could be useful in providing muscle relaxation during general anesthesia. The first report of the agent being used for this purpose occurred in 1942 by Dr. Harold Randall Griffith and his resident, Dr. Enid Johnson, during an appendectomy [1]. The synthesis of the alkaloid agent tubocurarine followed and its use during anesthesia began to gain traction. Further studies by Drs. John Halton and Cecil Gray with tubocurarine in 1946 laid the foundation for development of what became known as the “Liverpool anesthetic technique” – the first example of the concept of balanced anesthesia. The technique became popular and the practice of using deep planes of anesthesia to avoid movement was largely abandoned [2–4]. Within the next 10 years, the use of neuromuscular blockade for anesthesia in people had spread throughout the world. Possibly owing to more limited access to mechanical ventilators, or because most intubations and surgeries can be performed in veterinary species without the need for muscle paralysis, the uptake on incorporating neuromuscular blocking agents (NMBAs) into veterinary anesthesia has been much slower and more limited than in human anesthesiology. The depolarizing agent succinylcholine was first used for canine anesthesia in 1952 [5] and in 1954, Hall and Weaver described the use of the nondepolarizing agent gallamine coupled with positive‐pressure ventilation as part of balanced anesthesia in the dog and cat [6]. Since those initial reports, a significant body of evidence relating to the effects, clinical use, monitoring, and antagonism of neuromuscular blockade in veterinary anesthesia has now accumulated. While our understanding of these agents has grown exponentially, NMBAs remain part of only a small proportion of all cases in veterinary anesthesia [7]. NMBAs exert their actions by interrupting transmission through the NMJ. The NMJ is composed of a presynaptic motor neuron and a postsynaptic skeletal muscle cell separated by the synaptic cleft (Fig. 26.1). The presynaptic cell originates in the spine and travels uninterrupted to the target muscle. It loses its myelin sheath when it approaches the synapse and branches to contact several muscle cells, composing what is known as a “motor unit.” This presynaptic cell synthetizes and stores ACh in vesicles; both a reserve and a readily available population of ACh vesicles are found within this cell. Depolarization of the motor neuron results in an influx of Ca2+ via voltage‐gated channels that triggers the mobilization of vesicles and the release of a large amount of ACh into the cleft. When two molecules of ACh interact with both α subunits of a nicotinic acetylcholine receptor (nAChR) located in the folds of the postsynaptic cell, a conformational change occurs within the receptor, allowing an influx of Na+ into the skeletal muscle cell. Depolarization of the endplate terminal triggers the activation of voltage‐gated Na+ channels that propagate the action potential along the cell, signaling the process for muscular contraction [8,9]. The interaction between ACh and the nAChR is very brief, with its effects terminating almost immediately. Free ACh is promptly hydrolyzed by the acetylcholinesterase enzyme into choline and acetic acid [10]. The sudden and drastic reduction in the concentration of ACh in the cleft favors the movement of ACh away from the nAChR. The amount of ACh released by the nerve is affected by the concentration of extracellular Ca2+. The absence of Ca2+ would result in the absence of ACh release and muscular contractions, while doubling the concentration of Ca2+ would result in a 16‐fold increase in the amount of released ACh. Under normal circumstances, only a fraction of the available vesicles of ACh are released; however, this fraction is more than sufficient for effective neuromuscular transmission. Moreover, only a small number of available nAChR need to be activated to cause maximal depolarization of the muscle cell. These redundancies in the process of neuromuscular transmission provide the NMJ with a substantial margin of safety and an immense reserve [11]. Pentameric nAChRs are found postsynaptically in the shoulders of the primary clefts, and presynaptically in the motor nerve. The postsynaptic receptor contains two α1 subunits, which are the binding sites of ACh (and NMBAs). The fetal and mature forms of this receptor vary by only one subunit: a γ subunit in the fetal subtype, and the ε subunit in the mature form. β1 and δ subunits complete the receptor. The fetal subtype, and a third subtype composed of α7 subunits, are found during development, and are replaced by the mature form soon after birth; the adult form remains for life. The fetal and α7 subtypes can also proliferate in abnormal situations that result in decreased muscle activity, such as poor innervation, prolonged immobilization, or increased muscle catabolism (e.g., inflammation). Opening time of the sodium channel and sensitivity to specific ligands are altered in these receptors. This explains in part why increased sensitivity to succinylcholine and decreased potency of nondepolarizing agents are observed in neonates or during abnormal states when other subtypes of the nAChR proliferate. Upregulation of receptors may also be involved in the differences observed during neuromuscular pathologies [12,13]. Presynaptic nAChR are also found in the NMJ. Through positive and negative feedback loops, presynaptic receptors control the mobilization and release of ACh. A negative feedback loop stops further release of the transmitter when the concentrations of ACh in the cleft are sufficiently high. In a complementary mechanism, a positive feedback loop signals the mobilization of reserve ACh toward the active zone, increasing the population of readily available ACh in preparation for the next stimulus. The latter mechanism may play a key role in the development of fade during repeated nerve stimulation when nondepolarizing NMBAs are used (discussed in the section on monitoring neuromuscular transmission). The depolarizing agent succinylcholine does not inhibit this receptor, explaining why no fade is observed when that agent is used [14]. Figure 26.1 Representation of the neuromuscular junction and its main components. See text for details. Neuromuscular transmission can be interrupted, producing paralysis, and thereby providing one of the main components of anesthesia (relaxation/immobility) without the need for excessively profound depression of the central nervous system. NMBAs do this through one of two main mechanisms: depolarization of the postsynaptic cell (depolarizing NMBA) or competitive antagonism of the nAChR (nondepolarizing NMBA). The effects of NMBAs are measured by quantitating the reduction in the evoked force of contraction (or a surrogate). Potency is determined by constructing a dose‐response curve, which is composed of the responses to several submaximal doses (Fig. 26.2A). From that relationship, the effective doses that produce 50% and 95% depression of force of contraction (ED50 and ED95, respectively) can be identified. The ED95 can be used to compare agents given at equipotent doses, or to compare the characteristics of multiples of the ED95. The onset time is the interval between administration and a predetermined effect, typically 95% depression. Duration of action (sometimes called “duration of clinical or surgical block”) is the time between injection and the return of 25% (sometimes 10%) of force of contraction. The recovery index is the time between 25% and 75% recovery of the evoked force of contraction and describes the slope (or speed) of recovery. Complete recovery of neuromuscular transmission requires more time and is reached when no residual weakness can be measured (Fig. 26.2B). Figure 26.2 A. A dose‐response curve illustrating responses to varying submaximal doses of an NMBA (simulated data). The depression in the magnitude (or height) of the single twitch (ST) is measured relative to its magnitude prior to NMBA administration. The effective dose producing 50% (ED50) and 95% (ED95) twitch depression can be calculated. Source: Dr. Daniel M. Sakai, with permission. B. An example of the time‐course of neuromuscular block after administration of a nondepolarizing NMBA (simulated data). Time is shown on the x‐axis and the magnitude of evoked responses for the first response (T1; black bars and line) to train‐of‐four (TOF) stimulation, and the TOF ratio (T4:T1; red line) are shown on the y‐axis. Onset time is the period between NMBA administration and maximal effect. Notice that the magnitude of T1 returns earlier than the TOF ratio, making it a less sensitive indicator of partial block. Nondepolarizing NMBAs exert their action through competitive antagonism of the nAChR receptors. The binding of a nondepolarizing NMBA with at least one α1 subunit of the nicotinic receptor prevents the conformational change produced by ACh. Therefore, Na+ influx, depolarization, and contraction do not occur. In general, nondepolarizing NMBAs are large, water‐soluble molecules, with relatively low volumes of distribution (typically 0.2–0.4 L/kg) that do not cross the placenta or the blood–brain barrier. No sensory depression results from their use, therefore, other components of anesthesia, such as hypnosis and analgesia, need to be provided by different means. Onset time for neuromuscular blockade after injection of nondepolarizing agents is relatively slow, requiring 2–5 min to reach full effect. Both the onset and offset are progressive phenomena and respond to the concentration of drug at the site of action, the NMJ. Peak effect occurs with some delay (typically 1 min) after peak plasma concentration is reached, as the drug is transferred into the NMJ. Similar to other anesthetic agents, onset time is affected by cardiac output, muscle blood flow, and distance from the heart to each muscle. Therefore, onset time will vary between muscles. Moreover, the sensitivity of different muscles to the effects of an NMBA varies, with the diaphragm being characteristically resistant. That is, both the speed of action and the magnitude of the effect may differ between muscle groups after a dose is administered. In general, less potent agents have shorter onset times than more potent drugs. Owing to the margin of safety inherent to the NMJ, a large proportion of receptors need to be occupied before transmission is interrupted and contraction is abolished. Blockade occurs faster when larger numbers of molecules are administered, which is the case for less potent NMBAs. For each agent, increasing the dosage of the agent will accelerate onset and prolong duration. The margin of safety associated with neuromuscular transmission has distinct clinical consequences. It is estimated that before any effect from an NMBA can be appreciated, approximately 70% of the nAChRs must be antagonized [15]. Therefore, a small dose that only interacts with a smaller proportion of receptors may not have a measurable impact on neuromuscular transmission and force of contraction. This observation is the basis for the priming technique, which was once popular when slower agents were commonplace. With this technique, a small and mostly clinically inconsequential dose of an NMBA is given during preoxygenation. After a few minutes, induction is performed followed by a larger dose of the NMBA. The intent is that the priming dose has already occupied a number of receptors without depressing the force of contraction. The second dose will produce a faster onset as it will act on “active” receptors. Onset time with a priming method produces faster paralysis than if the complete dose was administered at once. A second consequence of the margin of safety is that the neuromuscular effects from an initial dose will begin to dissipate while a large number of nAChRs are still occupied by the drug. A second dose, administered to prolong the duration of neuromuscular block, will therefore produce a larger and longer‐lasting effect than the initial dose. This explains why anesthesiologists typically re‐dose an NMBA with aliquots that are only a fraction (commonly 1/3 to 1/2) of the initial dose. During offset, force of contraction is gradually restored until full neuromuscular transmission has recovered. Recovery occurs as the NMBA leaves the NMJ, a process closely related to a decay in plasma concentrations. Plasma concentrations will decrease because of redistribution, biotransformation, and excretion or, in particular cases, when binding agents are administered and the NMBA molecules are altered. Nondepolarizing NMBAs are classified into two main chemical families: (1) benzylisoquinoliniums (e.g., atracurium, cisatracurium, and tubocurarine), and (2) aminosteroids (e.g., vecuronium, rocuronium, and pancuronium) (Table 26.1). In general, benzylisoquinolinium compounds are intermediate‐acting NMBAs, with an expected duration of neuromuscular block of approximately 20–40 min. Agents of this family undergo extrahepatic paths of metabolism and most compounds are capable of releasing histamine upon injection. Aminosteroid compounds, on the other hand, do not generally cause histamine release and have fewer cardiovascular effects, although some agents may have a vagolytic effect leading to increased heart rate. Agents of this family undergo biotransformation and elimination via the liver or kidneys and, hence, clearance is altered when organ function is decreased. Modern agents in this family are largely considered intermediate‐acting drugs with pancuronium (typically classified as long‐acting) being an exception. Table 26.1 Selected neuromuscular blocking agents (NMBAs) used in veterinary medicine. Source: Compiled from Lee et al. [17]; Wright et al. [18]; Martin‐Flores et al. [19]; Cottrell et al. [20]; Martin‐Flores et al. [21]. a Values listed for the nondepolarizing agents are based on canine studies. Atracurium is a benzylisoquinolinium compound comprising a mixture of 10 isomers of varying potency that undergoes enzymatic degradation by nonspecific esterases, which accounts for approximately two‐thirds of its biotransformation [16]. These enzymes are distinct from acetylcholinesterase and plasma cholinesterase (also known as “pseudocholinesterase”). Hence, abnormal plasma cholinesterase concentrations do not affect degradation of atracurium. In addition, nonenzymatic degradation occurs spontaneously in the presence of normal pH and temperature via a process named “Hoffman elimination.” Decreases in temperature and pH will delay nonenzymatic degradation, although it is unlikely that changes in pH observed under most clinical situations will have a noticeable impact. Moreover, changes in pH may also affect enzymatic hydrolysis in the opposite direction. Therefore, small changes in pH are unlikely to result in clinically relevant changes in duration of action of atracurium. For storage, atracurium is provided in a low‐pH solution and should be kept refrigerated. Degradation of atracurium by either pathway results in the production of laudanosine [22]. This byproduct has no neuromuscular blocking effects but can be a central nervous system stimulant. High concentrations of laudanosine can increase the minimum alveolar concentration of volatile anesthetics by as much as 30% [23]. Laudanosine elimination occurs mostly through bile (~70%) and urine (~30%) and, unlike its precursor atracurium, is dependent on hepatic function [24]. Therefore, hepatic disease is unlikely to substantially affect the duration of atracurium but can result in accumulation of laudanosine. As an example, laudanosine, but not atracurium, has been shown to accumulate during the anhepatic phase of liver transplant in pigs [25]. Like most benzylisoquinolinium relaxants, including tubocurarine, atracurium can release histamine upon administration, which may cause tachycardia and arterial hypotension. However, this is typically observed after several multiples of the ED95 are used [26]. Cisatracurium is one of the isomers that compose atracurium. It was developed in an attempt to decrease the risk of histamine release and improve atracurium’s margin of safety. In humans, eight times the ED95 needs to be administered before clinical consequences of histamine release are observed; therefore, histamine release does not occur under normal clinical situations. Like atracurium, cisatracurium is degraded via Hoffman elimination. Nonspecific enzymatic hydrolysis occurs at substantially lower rates than for atracurium [27]. As in the case for atracurium, laudanosine is a byproduct of metabolism; however, smaller quantities are formed as cisatracurium is more potent than atracurium so smaller doses are used to produce equivalent effects [28]. Like atracurium, duration of action is considered intermediate; however, since cisatracurium is more potent than atracurium and fewer molecules need to be administered, onset is slower. Other examples in the benzylisoquinolinium family include the historically relevant agent tubocurarine (also known as “(+)‐tubocurarine” or “d‐tubocurarine”) and mivacurium. Tubocurarine was considered a long‐lasting agent that could release histamine at doses below the ED95, hence, cardiovascular effects were common with its use. Mivacurium was introduced to clinical practice as an agent of short duration, owing to its rapid hydrolysis by plasma cholinesterase (pseudocholinesterase), a degradation path shared with succinylcholine [29]. Consequently, abnormally low levels of this enzyme increase the duration of action of this agent [30]. While mivacurium’s effects are short‐acting in people, differences in enzymatic activity in dogs result in long‐lasting neuromuscular block in this species [31]. Like other agents of this family, mivacurium causes a dose‐dependent release of histamine. Of the aminosteroid NMBAs, pancuronium is longer‐lasting, with a duration approximating or exceeding an hour [17]. Owing to its vagolytic effect, administration of pancuronium can result in increases in heart rate and arterial blood pressure [32,33]. Vecuronium results from demethylation of pancuronium and, unlike the parent compound, is not vagolytic and is largely devoid of any cardiovascular effects. An active (3‐hydroxy) metabolite of vecuronium is produced during spontaneous deacetylation, which conserves 60–80% of the neuromuscular blocking potency. Vecuronium and its active metabolites are biotransformed by the liver and kidneys. Therefore, prolonged administration in the presence of decreased organ function is likely to result in accumulation [18]. Like vecuronium, rocuronium is an intermediate‐acting NMBA, but with a lower potency and faster onset. At equipotent doses, rocuronium has a faster onset than vecuronium, mivacurium, and cisatracurium [34]. Metabolism of rocuronium is limited, and most of the drug is excreted unchanged through urine and bile. Like vecuronium, no hemodynamic alterations are observed at clinically useful doses (up to four times the ED95) [35]. Succinylcholine is the only member of this family that is available for clinical use. The mechanism of action of succinylcholine is almost diametrically opposite to that of the nondepolarizing agents: succinylcholine binds to the nAChR and acts as an agonist. While the detailed mechanism of action is not fully understood, some key steps are known. Like ACh, succinylcholine binds the nAChR and depolarization of the membrane occurs. This opens voltage‐gated Na+ channels on the skeletal muscle cell resulting in muscle contraction. Closure and inactivation of the Na+ channels only occurs when the membrane potential is reset. Normally, with ACh acting as the agonist at the nAChR, this is a very rapid process as ACh is hydrolyzed by the acetylcholinesterase enzyme in the synaptic cleft. Succinylcholine, however, is not metabolized by this enzyme and the drug remains at the NMJ for far longer. The Na+ channels remain inactivated, transmission is blocked, and the muscle becomes flaccid. This depolarization‐induced block (also called “Phase I block”) is often preceded by an initial disorganized contraction of muscles (called “fasciculations”) before relaxation follows [36]. Fasciculations typically last for a very short period, although sustained contraction (several minutes) has been observed in the masseter and adductor pollicis muscles in humans [37]. Recovery from depolarization or Phase I block induced by succinylcholine occurs as the drug redistributes out of the NMJ, a process that is facilitated by the decrease in the plasma concentration as the agent is hydrolyzed by the enzyme plasma cholinesterase (also known as “pseudocholinesterase”) [36]. The enzyme is synthetized in the liver and, consequently, liver diseases may lead to lower levels of this enzyme and an increased duration of action of succinylcholine [38]. Abnormal plasma cholinesterase is present in individuals with a genetic mutation that affects the synthesis of the enzyme. In those cases, the duration of action of succinylcholine can be increased from an expected 10 min to 4 h [39]. A transfusion with whole blood or fresh frozen plasma might be required to terminate the effects of succinylcholine under those conditions. The activity of plasma cholinesterase can be assessed in suspect individuals by measuring the dibucaine number. Dibucaine is a local anesthetic that inhibits the activity of the plasma cholinesterase enzyme. The activity of the abnormal form of the enzyme is resistant to this inhibition. The dibucaine number indicates the percentage of enzyme activity that is inhibited when exposed to dibucaine and shows whether normal or atypical plasma cholinesterase is present. Under normal conditions in humans, succinylcholine produces a very fast onset and a short duration of action. There is species variation to these characteristics. A similar time‐course is seen in cats; however, a longer duration (20–30 min) is commonly seen in dogs, likely due to lower enzymatic activity [19,40]. When the NMJ is exposed to increasing doses of the drug, usually through repeated boluses or continuous infusions, succinylcholine‐induced block begins to assume the characteristics of a nondepolarizing (i.e., competitive) block and this phenomenon has been variably referred to as “Phase II,” “desensitization,” or “dual” block [36]. Unlike the nondepolarizing drugs, succinylcholine has several important effects on other organs. Interactions with muscarinic receptors can produce bradycardia, but interactions with sympathetic ganglia can increase circulating levels of catecholamines and result in tachycardia and hypertension. While no direct effect on the central nervous system occurs, increased intracranial and intraocular pressure have been reported [20,41–43]. Histamine release is possible following administration of this agent, and hence, swings in blood pressure should be expected. Hyperkalemia is also induced by administration of succinylcholine and may be fatal in individuals with muscle trauma or motor neuron defects [44,45]. Despite these drawbacks, succinylcholine remains in clinical use for humans, primarily (if not exclusively) due to its rapid onset. When a rapid sequence induction is necessary, succinylcholine produces acceptable intubating conditions faster than any other NMBA. Fumarate (olefinic isoquinolinium diester) compounds constitute a new family of nondepolarizing agents that are being developed. Several agents have been investigated, which provide either fast onset and ultra‐short duration, or intermediate duration that can be shortened by a novel mechanism of reversal. While all agents have a potential for histamine release, this does not occur at clinically useful doses [46]. Gantacurium offers a fast onset and very short duration. In cats, gantacurium had an onset time of 1–1.5 min and a duration of 4–7 min to complete recovery [21]. This short duration is the consequence of different paths of metabolism: a slow pH‐sensitive ester hydrolysis and a faster unique mechanism involving adduction of cysteine to the NMBA molecule. The adduction of cysteine occurs at the central fumarate bond, replacing chlorine. The resultant molecule has negligible neuromuscular blocking properties. This method of inactivation can be further accelerated by the administration of exogenous cysteine (L‐cysteine) [47,48]. Analogues of gantacurium (CW 002 and CW 011) have also been synthesized to undergo slower cysteine adduction, yielding an intermediate duration of action. Predictably, their effects can be substantially shortened by the administration of L‐cysteine [49]. Moreover, a newer agent (CW 1759‐50) that is rapid‐acting with a short duration like gantacurium, but with an improved cardiovascular profile, is also being studied [50]
26
Neuromuscular Blockade
History of neuromuscular blocking agents
Neuromuscular blocking agents in veterinary anesthesia
Anatomy and physiology of the neuromuscular junction
Neuromuscular blockade
Pharmacology and nomenclature
Nondepolarizing neuromuscular blocking agents
Drug
Onset (min)a
Duration (min)a
Elimination
Intravenous dose (mg/kg)
Intravenous infusion (mg/kg/h)
Nondepolarizing agents
Benzylisoquinoliniums
Atracurium
3–5
20–40
Ester hydrolysis, Hoffmann elimination
Canine 0.1–0.2
Feline 0.1–0.25
Equine 0.07–0.15
Canine 0.18–0.36
Equine 0.17
Cisatracurium
3–5
30–40
Hoffmann elimination
Canine 0.075–0.3
Feline 0.05–0.3
Aminosteroids
Vecuronium
3–5
30–40
Hepatic metabolism biliary and renal excretion
Canine 0.05–0.1
Feline 0.025–0.1
Equine 0.1
Rocuronium
1–2
20–30
Hepatic uptake and biliary excretion
Canine 0.1–0.6
Feline 0.1–0.6
Equine 0.3–0.6
Canine 0.2
Pancuronium
5
30–60
Hepatic metabolism and renal elimination of active metabolites
Canine 0.07–0.1
Feline 0.06–0.1
Equine 0.12
Fumarate diesters
Gantacurium
1–2
5
Ester hydrolysis, cysteine adduction
Canine 0.06
Feline 0.06
Depolarizing agent
Succinylcholine
1–2
6–20
Hydrolysis by plasma cholinesterase
Canine 0.3–0.4
Feline 0.1–0.2
Equine 0.12–0.15
Depolarizing neuromuscular blocking agents
Novel nondepolarizing agents
Stay updated, free articles. Join our Telegram channel
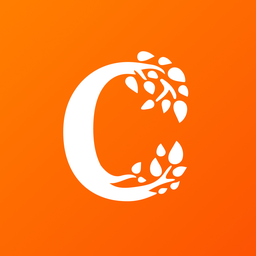
Full access? Get Clinical Tree
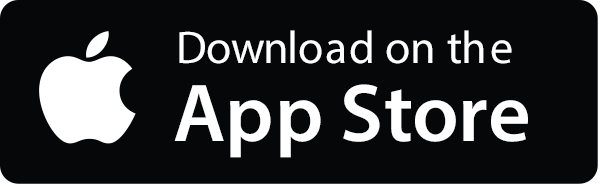
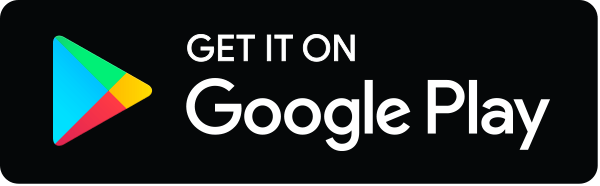