Erin Wendt‐Hornickle and Daniel Almeida Veterinary Clinical Sciences Department, College of Veterinary Medicine, University of Minnesota, St. Paul, MN, 55108, USA Patients with diseases involving the nervous system often require anesthesia for diagnostic imaging, sample collection, or treatment of the disease pathology. Others will require anesthesia for procedures unrelated to the disease of the nervous system. Understanding the physiology and pathology of the nervous system is important when considering an anesthetic plan during which the goal should be optimization of cerebral blood flow (CBF) and perfusion and prevention of increases in intracranial pressure (ICP). Anesthesia of patients with some neurologic conditions may be technically challenging and clinically complex. The requirement of advanced knowledge and specialized equipment may make some of these cases better suited for a clinical setting with an anesthesiologist. Cerebral blood flow is regulated by several factors, including the cerebral metabolic rate of oxygen (CMRO2), arterial partial pressure of carbon dioxide (PaCO2), and arterial partial pressure of oxygen (PaO2). The CMRO2 is proportional to cerebral (neuronal) activity; CBF needs to match metabolic requirements and it does so by the mechanism of flow–metabolism coupling. Acutely, PaCO2 exerts a significant influence on CBF, where flow varies directly with PaCO2 (each 1 mmHg change in PaCO2 causes CBF to change 1–2 ml 100 g−1 min−1). Those changes are due to rapid diffusion of CO2 across the cerebrovascular endothelium and consequent pH changes in the extracellular fluid of the brain. Although PaO2 exerts an influence in CBF, it is minimal until the PaO2 falls below 50 mmHg at which point CBF increases [1]. Hemodynamically, it is also helpful to understand CBF in light of Ohm’s law, where blood flow to the brain is a dependent variable and cerebral perfusion pressure (CPP) and cerebral vascular resistance (CVR) are the independent variables that control flow [2]. The brain receives a significant share of cardiac output considering the total tissue volume; in humans, the brain accounts for 2% of total body weight and receives 12–15% of total cardiac output [3]. In clinically normal canines, the CBF is approximately 67–90 ml min−1 100 g−1 tissue [4–6]. In clinically normal felines, the CBF is approximately 40 ml min−1 100 g−1 tissue [4, 5, 7]. The brain is unique in that an intact, bony, noncompliant skull encases the intracranial structures. All intracranial structures (tissue, cerebrospinal fluid [CSF], and blood) must have a fixed volume to maintain normal intracranial volume. Any increases in the volume of tissue, CSF, or blood without a concomitant decrease in the volume of another constituent will lead to an increase in intracranial volume and therefore an increase in ICP. This is illustrated by the Monro–Kellie doctrine (Eq. (3.1)) [8, 9]. The total volume (V) of intracranial constituents is equal to brain volume, CSF volume, and blood volume combined: If ICP continues to increase, herniation of brain tissue from its normal position within the skull may occur. Signs of brain herniation may include increasing systolic and pulse pressures, bradycardia, and irregular breathing, collectively known as the Cushing reflex [6, 9]. Efforts at preventing and treating increased ICP should be aimed at decreasing the volume of the intracranial constituents. This can be achieved with therapeutic interventions such as hyperventilation, hyperosmotic therapy, or diuretics. Though measurement of CBF is not commonly employed in veterinary medicine, there are both noninvasive and invasive methods described in human patients. Noninvasive techniques include ultrasonography of optic nerve sheath diameter, transcranial Doppler (TCD) ultrasonography, and near‐infrared spectroscopy (NIRS). TCD ultrasonography measures linear CBF velocity and pulsatility index within the intracranial arteries. NIRS is based on the differences in absorption of near‐infrared light by oxyhemoglobin and deoxyhemoglobin in cerebral tissue, reflecting the mixed venous–capillary–arterial oxygenation state of the brain tissue in the location of interest. Invasive methods of CBF include jugular venous oximetry, brain tissue oxygen monitoring, cerebral microdialysis, external ventricular drain, intracranial ventricular pressure measurement, and laser Doppler or diffusion flowmetry. A full review of CBF measurement modalities is beyond the scope of this chapter; however, there are many available resources reviewing the mechanisms of these measurement tools [10–12]. Autoregulatory mechanisms are those that help maintain the internal environment of the central nervous system (CNS), which can include hemodynamic responses and flow–metabolism coupling. The mechanism described here is the hemodynamic response which helps maintain adequate CBF over a range of perfusion pressures. It was long believed that under normal conditions CBF was strictly autoregulated, keeping it constant over a wide range of CPPs and mean arterial pressures (MAPs) between 60 and 140 mmHg (Figure 3.1). It is possible, however, that the lower limit of CBF autoregulation may vary between individual patients. Data in humans point to a higher limit for autoregulation, suggesting an MAP range of 70–150 mmHg; in patients undergoing cardiopulmonary bypass, the lower MAP limit of CBF autoregulation ranged from 40 to 90 mmHg [14]. Typically, during autoregulation when systemic pressure increases, cerebral capillary, arteriolar, and arterial constriction occurs, and when systemic pressure decreases, cerebral capillary, arteriolar, and arterial vasodilation occurs [4]. Once the ability to mount appropriate vasoactive responses is exhausted, CBF becomes completely dependent on changes in CPP [2]. Vascular responses are mediated by several cellular mechanisms, including nitric oxide, vasoactive peptides, potassium channels, and prostaglandins, as well as by markers of metabolic demands of neurovascular units (i.e., glutamate, adenosine, vasoactive intestinal peptide, and hydrogen and potassium ions). Several disease processes, including hypertension, intracranial space‐occupying lesions, traumatic brain injury (TBI), and the dose‐dependent presence of volatile anesthetics, affect autoregulation. Thus, blood flow patterns may be altered by the disease process itself in addition to general anesthetics. Figure 3.1 Impact of intracranial pressure (ICP), partial pressure of oxygen (PaO2), partial pressure of carbon dioxide (PaCO2), and mean arterial pressure (MAP) on cerebral blood flow in milliliters per 100 g of brain tissue per minute [4, 13]. Source: Canine and Feline Anesthesia and Co‐Existing Disease, 2015, with permission. As previously mentioned, as an independent variable, CPP has a large effect on CBF. CPP is defined as the difference between MAP and the higher of either ICP or central venous pressure (CVP) (Eq. (3.2)): In healthy dogs, ICP is 5–10 mmHg and CPPs are approximately 70–85 mmHg [15]. Although subtle species differences in these values may exist, the concept remains as follows: when ICP increases, MAP must also increase to maintain CPP. Since hypotension is common during anesthesia, low MAP must be monitored vigilantly and treated aggressively. Typically, the CBF and CMRO2 are directly related (i.e., under physiologic conditions, flow and metabolism are coupled). As regional CMRO2 increases or decreases, CBF changes in the same direction to meet metabolic requirements. The CMRO2 is influenced by several factors, including temperature and anesthetic drugs such as inhalants, sedatives, and analgesics [4]. Flow–metabolism coupling is critical in stressful physiologic conditions, such as hypothermia, hypotension, and hypoxia, which are not uncommon complications in small‐animal anesthesia. In humans, intravenous anesthetic agents seem to preserve CBF–CMRO2 coupling better than inhalant anesthetics [2]. The PaCO2 rapidly affects CBF; therefore, CO2 is often targeted in the treatment of increased ICP. Changes in PaCO2 will result in directionally proportional changes in CBF (Figure 3.1); CBF increases or decreases by approximately 2 ml min−1 100 g−1 of brain tissue for every 1 mmHg increase or decrease in arterial carbon dioxide within the range of PaCO2 from 20 to 80 mmHg [16]. This is mediated by the changes in the pH of the CSF surrounding the arterioles. The pH decrease with hypercapnia results in cerebral vasodilation, increased CBF, and increased ICP. Thus, it is important to maintain normocapnia (PaCO2 is approximately 35 mmHg, end‐tidal CO2 is approximately 30–35 mmHg) to prevent or treat cerebral vasodilation. Normocapnia is achieved through mechanical ventilation. Controlling PaCO2 can be instrumental in controlling cerebral blood volume and ICP in the short term. The PaO2 has an effect on CBF as well, but not until levels are below 50 mmHg (Figure 3.1). At this level of hypoxemia, cerebral vasodilation will occur, increasing CBF and ICP. Although monitoring oxygenation with arterial blood gases is the definitive way to accurately determine PaO2, it may not be available. As such, pulse oximetry can be used as a surrogate with the understanding that recognition of hypoxemia will be delayed while on 100% oxygen, according to the oxygen–hemoglobin dissociation curve [17]. Blood flows continuously out of the brain when ICP is higher than CVP. Under normal conditions, CVP has almost no effect on CBF. In situations wherein CVP is increased, however, CBF will also increase. Some conditions that may increase CVP include poor right ventricular contractility, increased venous return due to venoconstriction or hypervolemia, tension pneumothorax, heart failure, tamponade, pleural effusion, mechanical ventilation, positive end‐expiratory pressure, pulmonary hypertension, and pulmonary embolism. While much is published about CBF, there is comparatively much less known about the specifics of spinal cord blood flow. Similar to the cerebral circulation, the blood flow to the spinal cord is under autoregulation within a range of MAPs. Additionally, both hypercapnia and hypoxemia increase spinal cord blood flow. In general, when assessing anesthetic drug effects on spinal cord blood flow, attention is better spent on the hemodynamic effects of those drugs until more is known about their specific effects on spinal cord blood flow. In humans, the spinal cord has significant collateral blood flow, which can represent a “double‐edged sword,” as it may be protective against ischemia in normal conditions but may also lead to spinal cord hypoperfusion in conditions of systemic hypotension such as general anesthesia, as blood gets shunted away. It is reported that high spinal cord injuries can also lead to changes in CBF by affecting both the autoregulatory response and neurovascular coupling [2]. For patients who are unstable before anesthesia, extracranial stabilization should be the priority. Optimizing hemodynamic parameters such as cardiac output and blood pressure by initiating adequate volume replacement is of utmost importance. Certain neurologic conditions may affect ventilatory centers; therefore, adequate oxygenation and ventilation must also be a priority. After the most life‐threatening problems have been addressed, intracranial stabilization should follow shortly. This includes maintaining normal CBF, CPP, and ICP. Hyperglycemia is a common sequela to head trauma and is significantly associated with the severity of injury in both dogs and cats [18]. Hyperglycemia in the face of head trauma leads to higher mortality rates and worsened neurologic outcomes [19–21]. Glucose levels above 180 mg dl−1, specifically, are associated with poorer outcomes in patients with brain injuries [22]. However, because glucose is essential to cerebral metabolism and mental alertness, levels below 60 mg dl−1 should be treated with glucose supplementation [23]. Measures should be taken to prevent iatrogenic increases in CVP and ICP. Harnesses, not neck leads, should be used for restraint during ambulation. Also, jugular phlebotomy and, therefore, occlusion should be avoided. During periods of jugular occlusion in healthy dogs, ICP elevated to an average of 30 mmHg with some dogs reaching ICPs above that [15]. It is currently not practical to measure ICP in veterinary patients; changes in clinical signs that suggest increases in ICP must be assessed before anesthesia and closely monitored during and after anesthesia. The measurement of intraocular pressure (IOP) has been investigated as a noninvasive surrogate for ICP. Several human studies suggest that IOP and ICP are positively correlated when measured simultaneously [24]. Given the ease of measurement and the implications that early diagnosis of elevated ICP can have, it seems a beneficial diagnostic tool to add to the overall clinical picture. Absent or abnormal pupillary reflexes, mentation, and ventilatory pattern changes may be appreciated on physical examination. The Cushing reflex is a result of increased sympathetic discharge to the peripheral vasculature and a reflex increase in parasympathetic discharge to the heart, respectively [9]. These events occur because of increasing ICP and happen to maintain cerebral perfusion. Physiologic changes seen during this response include an increase in systemic blood pressure followed by a decrease in heart rate. Current recommendation in human post‐craniotomy patients and those with a history of head injury is to place the head in an elevated position relative to the heart and body. A head elevation of 30°–45° decreased and helped prevent further increases in ICP, respectively, by encouraging venous outflow [25–29]. In awake, healthy dogs, a head‐down position increased ICP by >100% compared to an elevated head position [15]. Veterinary patients with intracranial hypertension would also likely benefit from head elevation. Hyperventilation, thus lowering PaCO2, is the most effective method for rapidly reducing ICP by decreasing CBF [30–32]. This response is maintained during both inhaled and intravenous anesthesia [33]. A common recommendation is to maintain the PaCO2 between 30 and 35 mmHg in patients with increased ICP [4]. Because this approach mechanistically requires changes in the pH of extracellular spaces and CSF, once buffering systems neutralize these, continued hypocapnia beyond several hours will result in alkalosis and will no longer be effective at decreasing CBF. Furthermore, continued hypocapnia can have deleterious effects on many other systems. It can cause cerebral ischemia, especially in areas of the CNS with a lower basal CBF [34]. It can worsen cerebral ischemia in cases of traumatic brain injuries [35]. Hypocapnia can also decrease perfusion to splanchnic organ and myocardial and musculoskeletal tissues. It affects coagulation by provoking an increase in adhesion and platelet aggregation. Hypocapnia can also cause acute lung injury and other less‐severe pulmonary changes such as bronchoconstriction and attenuation of hypoxic pulmonary vasoconstriction [35]. It is not currently recommended in humans to hyperventilate patients prophylactically, as it will not prevent increases in ICP, nor is it recommended to abruptly discontinue hyperventilation, as rebound increases in CBF may develop [34]. For these reasons, maintaining a low‐normal PaCO2 (approximately 35 mmHg) is recommended for veterinary patients. The evidence that hyperosmotic therapy improves outcomes in patients with neurologic injury is limited. The most common hyperosmotic agents administered are mannitol and hypertonic saline. Mannitol is an osmotic diuretic and may be used to reduce ICP in patients with elevated ICP [36]. Mannitol increases plasma osmolarity, theoretically causing a movement of water down an osmotic gradient from the intracellular and interstitial areas of neuronal tissue to the intravascular space within the brain and ultimately to the systemic circulation. Mannitol may also reduce ICP by increasing cerebral perfusion, causing cerebral vasoconstriction [37, 38], and by decreasing the production of CSF [39]. Mannitol’s effects peak around 30–45 min after administration and last roughly 6 h [40]. Despite its many mechanisms of ICP reduction, mannitol may cause elevations in ICP through reversal of the initial plasma‐to‐blood osmotic gradient in patients without an intact blood–brain barrier [41], or through acute expansion of the intravascular fluid volume causing an elevation in CVP. In these cases, hypertonic saline may be a viable alternative. Hypertonic saline works similarly to mannitol, causing a net loss of water from intracranial structures to the systemic circulation. It may be used in patients with intracranial pathology to reduce or prevent increases in ICP [42–44]. In cases of TBI, hypertonic saline is more effective than mannitol in treating elevated ICP [45–48]. In humans with traumatic brain injuries, administration of hypertonic saline correlated with reduced intracranial hypertension in addition to improving mortality and neurologic outcome [49]. Hypertonic saline’s effect on decreasing intracranial volume, however, may be limited to <90 min [50], limiting its use for long‐term control of elevated ICP. Recently, the COBI (continuous hyperosmolar therapy for traumatic brain‐injured patients) trial was being conducted, which may shed more light on the outcomes of patients receiving hyperosmotic therapy post TBI [51]. Acepromazine has historically been implicated in decreasing the seizure threshold in patients with a history of seizure activity. A retrospective study has since indicated that acepromazine can be used without the risk of increasing the likelihood of seizure [52]. Since acepromazine exerts antagonist effects on alpha‐1‐adrenergic receptors leading to vasodilation and subsequent hypotension, this sedative should be used with caution in patients with intracranial or spinal pathology (Table 3.1). MAP should be closely monitored to maintain CPP (Eq. 3.1). Dexmedetomidine is an excellent choice in patients with intracranial disease, provided systemic hypotension is avoided. Dexmedetomidine decreases CBF in dogs anesthetized with halothane or isoflurane [53, 54]. Animal and human studies indicate that there is likely a neuroprotective effect in part through reduction of neuroendocrine hormones and inflammatory mediators such as tumor necrosis factor‐alpha (TNF‐α) and IL‐6 [55]. In addition, dexmedetomidine also significantly reduces postoperative cognitive dysfunction when compared to other sedatives [55]. Midazolam is a reasonable choice for sedation of neurologic patients. Midazolam reduces CBF by decreasing the CMRO2 consumption; [56] additionally, midazolam is effective as an anticonvulsant. Caution should be exercised, as benzodiazepines may cause paradoxical excitement when used as a preanesthetic agent. Opioids are an acceptable choice for premedication and analgesia in neurologic patients, as they have minimal direct effects on CBF and ICP [57]. Opioids, however, can indirectly increase ICP, as administration may cause emesis [58, 59], which can cause increases in ICP. They can also cause hypoventilation, leading to an increase in ICP secondary to hypercapnia; therefore, assisted, or mechanical ventilation during general anesthesia is recommended [60]. Because pain can also cause respiratory depression, avoiding the use of opioids as analgesics in these situations cannot be recommended. Further exploration into alternatives to opioid analgesia may allow for an eventual decrease in opioid use. Barbiturates, etomidate, propofol, and alfaxalone all decrease CBF and ICP and contribute to the decrease in CMRO2 seen with CNS depression [61–65], and have all been used in patients with neurologic injury. Historically, thiopental blocks sodium, potassium, and calcium flux, scavenges free radicals, blocks seizures, and improves regional blood flow [66]. Because of the negative effects on cardiovascular physiology and immune function, barbiturates are typically reserved for patients with refractory increases in ICP. Though etomidate decreases CMRO2, it has not been proven to provide neuroprotection or improve outcomes in cases with cerebral ischemia. In addition, etomidate can cause retching and myoclonic muscle activity, potentially increasing ICP. Propofol is the current standard for control of ICP in human intensive care units and is an appropriate choice for anesthesia in veterinary patients with intracranial pathology. At this time, the evidence for using the current formulation of alfaxalone in human or veterinary patients under these circumstances is limited. Table 3.1 Effects of opioids, sedatives, and anesthetics on intracranial pressure (ICP). a Can indirectly increase through hypoventilation or emesis b May cause increases secondary to retching c May cause no change if used with other sedatives and controlled ventilation Ketamine use in patients with intracranial pathology is slowly becoming more favorable. Unlike other injectable anesthetics, ketamine does not reduce CBF, ICP, or CMRO2 [13]. Early studies examining ketamine’s effect on intracranial hemodynamics were performed in humans without concurrent sedation or mechanical ventilation. However, the use of ketamine in patients with controlled ventilation has demonstrated no increase in ICP [67]; the use of other sedative agents will also blunt increases in CBF caused by ketamine. Therefore, with the use of mechanical ventilation and its use combined with sedatives/muscle relaxants, ketamine may be a reasonable choice in veterinary patients with intracranial pathology. An analysis of human TBI literature [68] demonstrated that not only did ketamine administration not increase ICP but that there were no adverse events associated with its use. In a retrospective study of human trauma patients receiving sedative agents, those that received ketamine had lower mortality rates after controlling for type of injury [69]. The effects of ketamine on N‐methyl‐D‐aspartate (NMDA) receptors and glutamate may impart a protective effect in patients with TBI. Intravenous lidocaine has been shown to be neuroprotective in experimental studies of hypoxia and ischemia. This seems to be effective when given prior to and shortly after the injury, limiting its clinical use. The mechanism of action is not entirely known; however, it is likely a combination of its effects on sodium channels, preservation of cellular mitochondria and adenosine triphosphate (ATP), and the reduction of neuroinflammation [70]. Total intravenous anesthesia (TIVA) is the sole use of injectable anesthetic agents for the maintenance of anesthesia. TIVA has been recommended for patients with intracranial pathology to prevent further increases in CBF and thus ICP. In humans undergoing anesthesia for the surgical treatment of acute subdural hematomas, propofol‐based TIVA provided better brain relaxation and maintained a lower ICP and better hemodynamics when compared to inhalational anesthesia [71]. Few disadvantages exist. They include cumulative drug effects, reliance on hepatic or other means of drug biotransformation, and the need for specialized equipment or proficiency in dosage and infusion calculations for adequate delivery. In general, inhalant anesthetics increase CBF and decrease CMRO2
3
Neurologic Disease
Introduction
Nervous System Physiology
Cerebral Blood Flow
Autoregulation
Cerebral Perfusion Pressure and Mean Arterial Pressure
Cerebral Metabolic Rate
Arterial Partial Pressure of Carbon Dioxide and Oxygen
Central Venous Pressure
Spinal Cord Blood Flow
Anesthesia of Patients with Neurologic Conditions
Preanesthetic Considerations
Glycemic Control
Other Nursing Strategies
Methods to Manage Increased Intracranial Pressure
Patient Positioning
Controlled Ventilation
Hyperosmotic Therapy
Anesthetic Management (Box 3.1)
Sedatives and Analgesics
Injectable Anesthetics
Drug or drug class
Effect on ICP
Opioids
Decreasea
Benzodiazepines
No change or decrease
Acepromazine
Potential increase secondary to cerebral vasodilation and increase CBF
Alpha‐2‐adrenergic agonists
No change
Propofol
Decrease
Etomidate
Decreaseb
Barbiturates
Decrease
Ketamine
Increasec
Isoflurane, sevoflurane, desflurane
In general, no change if <1 MAC
Increase if >1 MAC or if hypercapnia occurs
Nitrous oxide
Profound increase
Inhalant Anesthetics
Stay updated, free articles. Join our Telegram channel
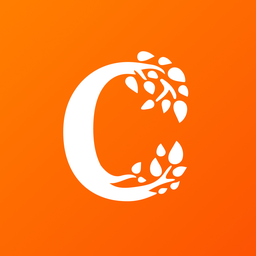
Full access? Get Clinical Tree
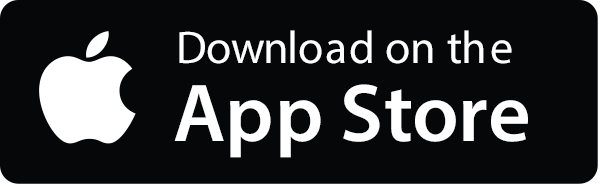
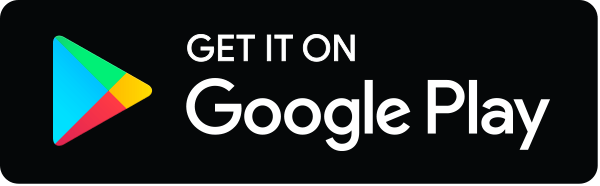