Fig. 1.
MR image weighting options in animal models of TBI. (a–c) Coronal images of a rat brain taken at 4.7 T, 2 days after midline fluid percussion injury. (a) T1-weighted MRI (TR = 500 ms; TE = 15 ms); (b) T2-weighted MRI (TR = 3,000 ms; TE = 80 ms); (c) T2*-weighted MRI (TR = 350 ms; TE = 10 ms; flip angle, 20°). (d) A corresponding H & E stained histological section from the same brain. Arrows point to hemorrhage in the corpus callosum. (e–g) Horizontal T2-weighted images of a mouse brain taken at 9.4 T, 2 days after controlled cortical impact injury. Arrows indicate tissue edema at the lesion site. (h–i) Coronal T2-weighted images (TR = 2,500 ms; TE = 45 ms) of a mouse brain taken at 9.4 T, 28 days after controlled cortical impact injury. The increased spatial resolution resulting from the higher magnetic field is apparent (compare to a–c). At 28 days, the T2-weighted images reveal enlarged lateral ventricles and the fluid-filled cavity that has developed at the injury site. An asterisk indicates cortical thinning ipsilateral to injury. Images are reproduced from Iwamoto et al. 1997 with permission from Wolters Kluwer and from Onyszchuk et al 2008 (28).
Both qualitative and quantitative evaluations of TBI pathology can be carried out using MR imaging. By measuring the area of a traumatic lesion on a series of MR images and multiplying by the image slice thickness, a good estimate can be made of the lesion’s total 3-dimensional volume. Lesion volumes calculated with this technique strongly correlate with histological estimates of lesion volume (1, 7, 8). However, using MRI to assess TBI lesions offers distinct advantages over histological methods. Fewer animals are required, since there is no need to sacrifice separate groups at each time point. Moreover, the potential variability inherent in a cross-sectional study design is reduced. Investigators can track the effects of TBI noninvasively as the lesion pathology develops. Perhaps more importantly, researchers can also follow the tissue effects of novel drug treatments or rehabilitative interventions in longitudinal studies of brain injury and recovery.
A key advantage of MRI in basic research is the potential for direct clinical comparison, since MRI scanning is commonly used in the clinical workup of TBI patients. Although other neuroimaging techniques such as CT are also used routinely in the clinic, the wide variety of image weighting options make MRI the most successful approach for detecting injury-related abnormalities in brain tissue. A further advantage is that MRI does not require the use of ionizing radiation or radioactive contrast agents.
The conventional MRI techniques introduced above yield primarily anatomical data that, when considered alone, provide limited functional insight into degenerative and recovery processes after TBI. However, this past limitation of MRI has been rapidly changing with the implementation of novel techniques and applications. Many of these newer MRI approaches have been relatively underutilized in experimental TBI research, but they offer considerable promise for future investigations.
Diffusion tensor imaging (DTI) is one specialized MRI technique with great potential for assessing brain injury mechanisms. DTI measures water diffusion in the brain with spatial mapping of the fractional anisotropy (a measure of how directional, or anisotropic, the diffusion is) and apparent diffusion coefficient (an estimate of the average magnitude of water movement). Interest in this imaging approach has grown in recent years, as DTI is more sensitive than conventional MRI to white matter injury. White matter changes observed with DTI after brain injury may reflect both axonal degeneration and reactive plasticity (9, 10).
Manganese-enhanced MRI (MEMRI) is another emerging MRI approach that allows visualization of white matter tracts and functionally connected circuits within the brain. After systemic or localized administration of manganese chloride, Mn2+ is taken up by active neurons through voltage-gated calcium channels and distributed via anterograde and retrograde axonal transport. Transynaptic exchange of Mn2+ also takes place between functionally connected neurons. Since Mn2+ is a paramagnetic agent that alters the appearance of T1-weighted MRI, the distribution of Mn2+ shows the white matter architecture. Decreases in the rate of transfer between connected regions can indicate neuronal dysfunction accompanying neurodegeneration (11). Thus, future studies could utilize MEMRI to assess white matter integrity and plasticity, neuronal activity, and axonal transport dynamics after TBI.
A third novel MRI approach is the emerging field of Molecular MRI, in which a specific molecule or drug, receptor, or cell type is tagged with a contrast agent so that its distribution may be visualized with in vivo imaging. Molecular MRI has been used for assessing the endogenous macrophage response after experimental TBI (12) and for in vivo tracking of transplanted stem cells after TBI (13). Future studies could make use of a new class of “responsive” contrast agents, which change relaxivity (and thus become detectable on MRI) in response to specific cellular or molecular events such as enzyme activity, changes in pH, or receptor binding (reviewed in ref. (14)). With the right contrast marker, MRI could in theory be used as an in vivo functional assay for nearly any event of interest in the pathological sequelae of TBI, making this a highly promising area for future research.
Dedicated research MRI systems for small animals are now available (Bruker Biospin; Varian Inc.) with magnet strengths typically ranging from 4.7 to 14 T. Some MRI systems allow simultaneous imaging of multiple animals for higher experimental throughput. Although greater magnetic field strength provides greater signal-to-noise ratio and improves the spatial resolution of images, high field strength systems require a large amount of dedicated laboratory space. Potential alternatives are the recently developed “benchtop” MRI systems, which are small and portable but offer more limited resolution at 1–1.5 T (MR Solutions; Stoelting).
3 Magnetic Resonance Spectroscopy
In addition to the structural and functional imaging approaches described above, MR provides a quantitative, noninvasive approach for measuring endogenous and possibly introduced neurochemicals. Similar to the hydrogen nucleus (proton) that is the source of the MRI signal, certain other naturally occurring nuclei also have a magnetic resonance signal. Of particular biological significance are carbon (13C), nitrogen (15N), and phosphorus (31P) nuclei. As described in the MRI section, resonant nuclei induce a signal in the scanner detector. In contrast to the spatial information encoded in MRI, MRS focuses on the discrete resonant frequencies of specific nuclei, which are dependent on the applied magnetic field strength. Each nuclear isotope has a characteristic frequency range. For example at 9.4 T, hydrogen (protons) resonate around 400 MHz. In comparison, carbon resonates around 100 MHz. In contrast to the anatomic data generated from MRI, magnetic resonance spectroscopic (MRS) data usually come in the form of a spectrum where the distinct frequencies correspond to individual neurochemicals within a discrete volume of tissue (voxel; see Fig. 2). Each peak in the spectrum represents an individual chemical environment. Proton MRS voxels in animal models are usually chosen to be small enough to fit within a single brain hemisphere, typically about 10 mm3. Although multivoxel acquisitions are possible and are commonly used in larger human brains, they are technically more challenging in animal models.
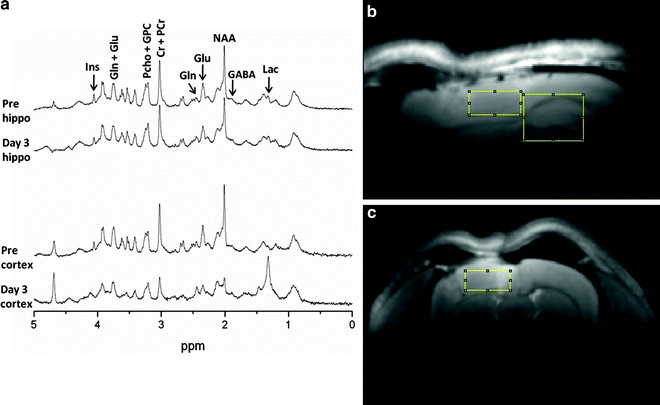
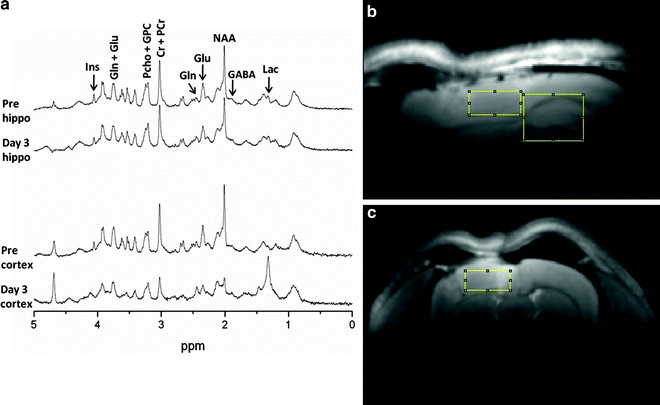
Fig. 2.
Representative proton MR spectra and voxel localization. A 9.4 T scanner was used to obtain MR spectra (TR = 4,000 ms; TE = 2 ms) from a 3 × 2.5 × 3 mm voxel over hippocampus, corpus callosum, and dorsal thalamus, and from a 2.7 × 1.3 × 2.7 mm voxel over cerebral cortex. Spectra were collected preinjury and then at 3 days after controlled cortical impact in rats. (a) The spectra show injury-induced changes in brain neurochemicals within both voxel locations. In hippocampal tissue that appears normal with conventional MR, NAA is reduced by 20% 3 days after TBI. In cortical tissue directly at the site of impact, NAA and Creatine are dramatically reduced (70% and 54%) while Lac is greatly increased after injury (14-fold). (b and c) T2-weighted MR images from 3 days after TBI showing cortical and hippocampal voxel locations. (b) Sagittal image showing cortical and hippocampal voxels. (c) Coronal image showing the cortical voxel. The hippocampal voxel is out of the plane of view. While using a surface RF coil optimizes sensitivity for MRS, it results in visible ventral signal dropoff in these images. Note edema in the injured cortex and downward shift of the corpus callosum from cortical swelling.
The earliest MRS experiments in animal models of TBI used 31P-MRS to quantify adenosine triphosphate, adenosine diphosphate, phosphocreatine (PCr), inorganic phosphate (Pi), and the free phosphomonoester and phosphodiester components of membranes. By calibrating the precise position (chemical shift) of the Pi peak within the spectrum, a noninvasive estimate of the intracellular pH can be obtained. Similarly, since ATP binds magnesium, estimates of the magnesium concentration in vivo can also be made following careful measurements of the chemical shift of the ATP resonances. Following fluid percussion injury, the PCr/Pi ratio has been shown to decline and the tissue was acidotic, although no changes in ATP, indicating impaired energy metabolism, were detected (15). Other studies have shown reduced intracellular Mg2+ following TBI that is attenuated by intravenous supplementation. Of potential clinical significance, Mg2+ concentration was correlated with postinjury performance on the rotorod (16).
Hydrogen MRS, also known as proton MRS, may be used to quantify numerous endogenous neurochemicals such as N-acetylaspartate (NAA), creatine, PCr, myo-inositol, scyllo-inositol, glutamine, glutamate, glucose, glutathione, lactate, pyruvate, taurine, and GABA. Proton MRS is rather more complicated than 31P-MRS, since the tissue water signal, which dominates the neurochemical signals by up to seven orders of magnitude, must be suppressed. Moreover, since skull and subcutaneous fat signals are extremely strong and can interfere with resonances of greater interest, MRS signals must be localized to discrete brain regions. Fortunately, sophisticated acquisition sequences are available on commercial MR instruments to achieve the necessary suppression rates for high-quality spectroscopy.
Since MRS, like MRI, is noninvasive, longitudinal studies are feasible. Studies by Schuhmann et al. (17) have shown that NAA, a neurochemical produced in the neuronal mitochondria, shows a postinjury time-course of changes similar to histological evidence of neuronal pathology. Similarly, choline and myo-inositol, putative markers of inflammation, followed similar time-courses to those of histological markers of inflammation. Other MRS-visible neurochemicals such as glutamate and glutamine, which are involved in the glutamatergic neurotransmission system and glutamate excitotoxicity, and glutathione, an important anti-oxidant species, are likely to be informative in pathological processes. Accordingly, proton MRS might be useful in explorations of cellular and molecular mechanisms of TBI. Proton MRS also has considerable translational value since it is widely available on commercial clinical scanners for studies in humans. Several studies have shown that MRS neurochemical biomarkers such as NAA and choline are strongly correlated with cognitive recovery following human TBI (18, 19).
Other nuclei that are of biological significance, such as 13C and 15N, also have MRS signals. These isotopes are low in natural abundance and their weak endogenous signals are relatively uninformative. However, it is possible to introduce metabolites that are enriched with these isotopes and carry out tracer studies to determine the metabolic fate of specific chemical moieties. Studies by Bartnik et al. demonstrated upregulated pentose phosphate metabolism after moderate to severe TBI by following the metabolism of [1,2-13C]-labeled glucose as the label is incorporated into glutamate, glutamine, and lactate (20). Although the spectroscopic acquisitions in these particular experiments were carried out in extracts, they are feasible in the living brain in vivo. Studies using 15N-labeled species, although to date not implemented in TBI, have the potential of following amino acid pathways noninvasively.
Magnetic resonance spectroscopy in general has certain advantages as a tool for metabolic imaging. First, acquisition sequences for proton MRS are implemented on MRI scanners using the same hardware configuration as MRI. Accordingly, MRS acquisitions can be completed in the same scanning session as an MRI study and the data sets are inherently registered in three-dimensional space allowing direct comparison between structure and metabolism. Moreover, compared to the radionuclide studies described below, labeled MRS studies do not require radioactive isotopes meaning that longitudinal studies on individual animals are feasible. Another advantage of isotopically-enriched MRS is that the metabolic fate of specific labeled nuclei can be followed as the labeled compound is metabolized over time. In contrast, the sensitivity of MRS is lower than the various forms of radionuclide imaging resulting in poorer spatial and/or temporal resolution. Finally, although radiolabeled analogs of many drugs are available for PET and SPECT tracer studies, the relatively low concentrations of drugs mean that analogous labeled studies using 13C, for example, are likely not feasible.
4 Micro-PET
Positron emission tomography (PET) is a functional imaging modality which tracks the localization of positron-emitting radioactive tracer molecules administered to the test subject. As the radioactive tracer decays, each emitted positron collides with an electron within the tissue nearby, producing 2 gamma rays traveling in opposite directions. These gamma rays are detected by an array of scintillation detectors surrounding the subject, and signals are fed to a computer, which localizes the sources of the gamma rays and combines these points into a 3-dimensional image. The term “micro-PET” refers to a high-resolution PET system designed specifically for imaging in small animal research models. The spatial resolution of micro-PET scanners currently approaches 2 mm.
< div class='tao-gold-member'>
Only gold members can continue reading. Log In or Register a > to continue
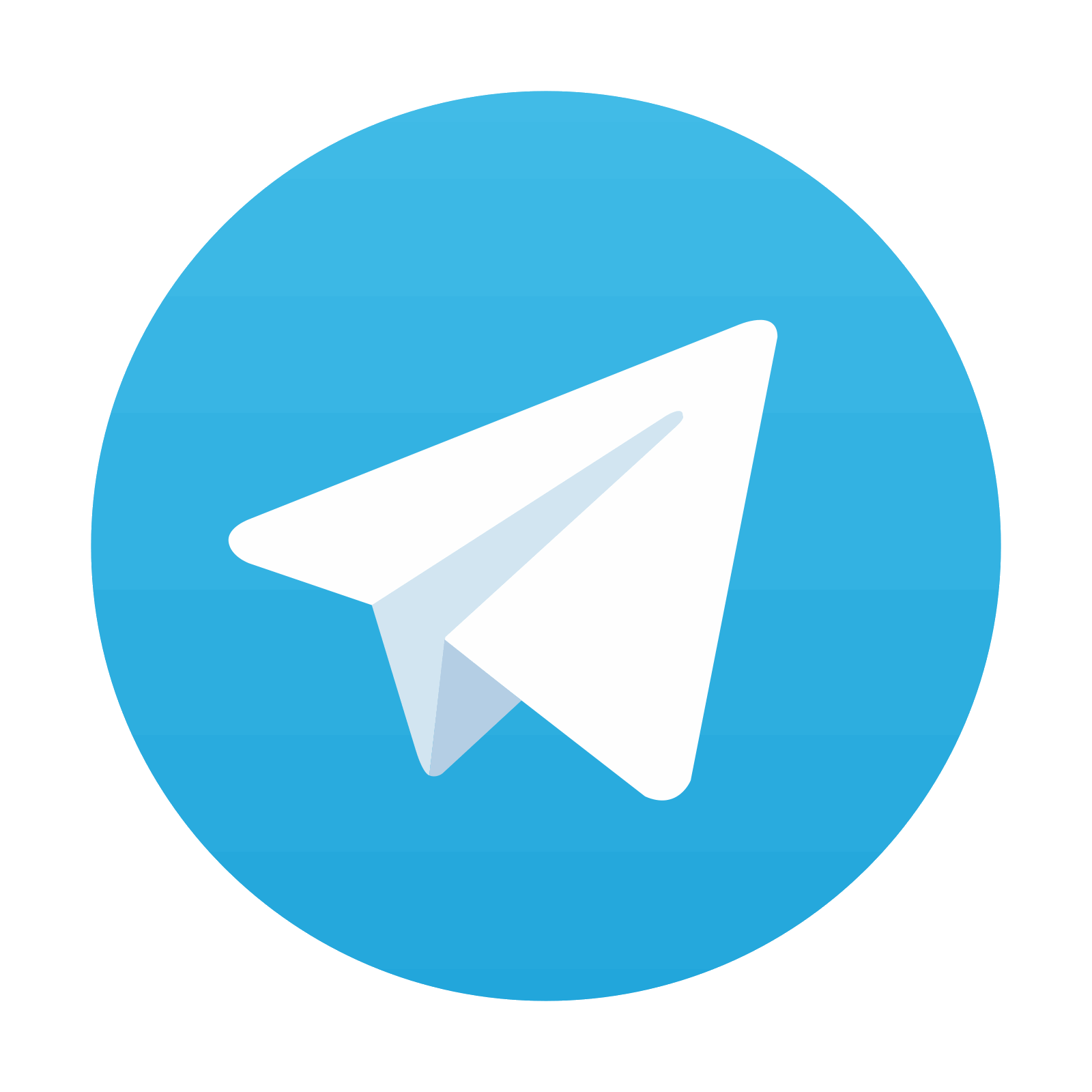
Stay updated, free articles. Join our Telegram channel
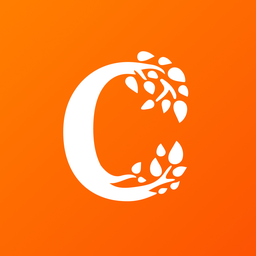
Full access? Get Clinical Tree
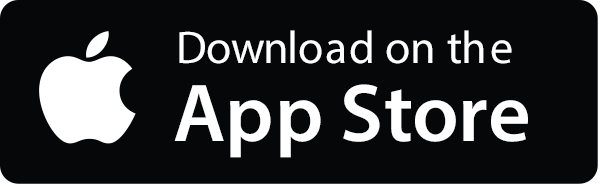
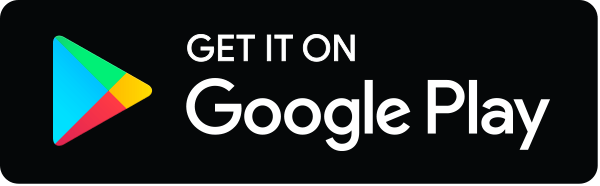