THOMAS F. FLETCHER
Nervous tissue parenchyma consists of neurons and supportive cells called neuroglia. Nervous tissue forms the nervous system, which may be divided into the central nervous system and the peripheral nervous system. The central nervous system (CNS) includes the brain and spinal cord. The peripheral nervous system (PNS) consists of cranial and spinal nerves, including associated nerve roots and ganglia. Nerves and ganglia that innervate viscera are designated the autonomic nervous system.
NEURONS
Neurons are the structural and functional units of the nervous system. They are also trophic units because often they transform and sustain what they innervate. Generally, neurons must last a lifetime because, with some exceptions (e.g., olfactory neurons and neuronal stem cells), mature neurons are incapable of mitosis. However, recent evidence of new neuron formation in several regions of the brain has been discovered.
Morphologically, neurons feature elongated processes, termed axons and dendrites, that extend variable distances from the cell body (perikaryon) (Fig. 6-1). Metabolically, neurons are actively involved in maintaining their structural integrity and in synthesizing, packaging, transporting, and releasing secretory products.
Neurons specialize in excitability and they communicate by releasing chemical agents (neurotransmitters, neuromodulators, or neurohormones). Excitation involves ions passing through protein channels embedded in neuronal plasma membrane that otherwise acts as a hydrophobic barrier to ion flow. Via chemical secretion, neurons transmit excitation to other neurons or to muscle or glands.
Neuronal Structure
Neurons assume a variety of shapes and sizes, related to their functional roles. Neuronal processes are configured according to the connections that must be made. Neurons with processes that extend long distances must necessarily be larger than less extensive neurons. Also, because larger processes conduct more rapidly than smaller ones, neurons that convey urgent information are large.
Cell Body
The cell body (perikaryon; soma) of a neuron consists of the nucleus plus the surrounding cytoplasm and plasma membrane (Fig. 6-1). In routinely stained histologic sections, the cell body is the most identifiable feature of a neuron. Cellular constituents are synthesized within the cell body and then flow distally into neuronal processes (axon and dendrites).
Cell bodies range from less than 10 µm to more than 100 µm in diameter. Cell body size is proportional to neuronal total volume, although the cell body itself represents a minor portion of the total volume (and an even smaller fraction of total surface area). In multipolar neurons, where the cell body plasma membrane integrates synaptic input, smaller cell bodies are more easily excited to threshold than larger ones; that is, less synaptic activity is required to trigger action potentials in small neurons (which have high input impedance, by virtue of their size).
Nucleus
Typically, the nucleus of a neuron is centrally positioned, spherical or ovoid, and relatively euchromatic, reflecting its high synthetic activity. Small neurons have rather heterochromatic nuclei. Neurons in autonomic ganglia have eccentric nuclei. Nuclear size is proportional to neuron size. The nucleus appears relatively large in a neuron because the cell body cytoplasm surrounding it represents only a minor fraction of total cell volume.
A prominent nucleolus is evident within the nucleus. In females of some species, sex chromatin (Barr body) may be evident in the vicinity of the nucleolus (cats, rodents) or nuclear membrane (primates).
Cell body cytoplasm
Many proteins are synthesized in the cell body cytoplasm of the neuron, including cytoskeletal proteins (e.g., for neurofilaments and microtubules), membrane proteins (e.g., for ion channels and active transport), enzymatic proteins (e.g., for glucose metabolism and neurotransmitter synthesis), and secretory peptides (e.g., neuromodulators and neurohormones).
The cell body cytoplasm of large neurons stained with aniline dyes and examined by light microscopy features clumps of chromatophilic substance (Nissl substance), which represent aggregations of rough endoplasmic reticulum (rER), free ribosomes, and polyribosomes. Chromatophilic substance extends into the trunks of dendrites, but it is absent from the axon and axon hillock, a pale-staining region of the cell body where the axon originates. Instead, the hillock contains neurofilaments and grouped microtubules. In small neurons, cytoplasmic chromatophilia appears relatively pale and diffuse.
FIGURE 6-1 Schematic illustration of a typical (multipolar) neuron, showing multiple dendrites and one axon emanating from the cell body. Functional regions include a dendritic zone (cell body and dendrites), which receives synaptic input; telodendritic zone (axon terminals), which makes synaptic contact with other neurons; and the axon, which conducts excitation between the two zones. This axon is drawn myelinated. The myelin insulation is interrupted by nodes (gaps). The myelin of each internode is formed by individual glial cells (not shown).

When a neuron is injured (e.g., by transection of its axon), the cell body swells, the nucleus shifts to an eccentric position, and ribosomes disperse so that chromatophilic substance disappears from the center of the cell body (chromatolysis). This response to injury, called the axonal reaction (Fig. 6-2), begins within days of the injury and may persist several weeks. The reaction is considered pathologic evidence of nervous tissue damage in the CNS.
Organelles in the cell body cytoplasm of the neuron include plentiful mitochondria, which support the aerobic energy needs of the cell, and a prominent Golgi complex. Secretory vesicles originate from the complex and are transported through the axon to synaptic boutons (expansions of the axon terminal). Secretory vesicles commonly contain neuroactive peptides that influence the excitability or growth of target cells. In the case of certain hypothalamic neurons, secretory vesicles contain neurohormones that are released in proximity to blood vessels and ultimately enter the bloodstream.
Synaptic vesicles containing neurotransmitters are concentrated in axon terminals, where they fuse with plasma membrane and release neurotransmitter into a synaptic cleft. Long-lived neurons may accumulate lipofuscin granules in the cytoplasm as a residue of lysosomal activity.
Microtubules (25 nm in diameter) and neurofilaments (10 nm in diameter) are numerous in the cell body. Microtubules are involved in the rapid transport of membrane-bounded organelles within the neuron. Groups of neurofilaments, referred to as neurofibrils, can be visualized with light microscopy.
Neuron Processes
A typical neuron has a single axon and multiple dendrites originating as processes from its cell body (Fig. 6-1). The axon ends in terminal branches that synapse with dendrites and cell bodies of other neurons or innervate muscle or glandular epithelium. Each type of neuronal process has a distinct functional role within the neuron. Thus, the processes have different populations of plasma membrane proteins (channels, receptors, transporters, pumps).
Dendrites
Dendrites are highly branched processes designed to receive numerous synaptic contacts from other neurons. Treelike, each dendrite emerges as a main trunk that branches repeatedly into smaller and smaller twigs. The trunk has an organelle content similar to that of the cell body. Small dendritic branches feature predominantly microtubules, augmented by neurofilaments, mitochondria, and smooth endoplasmic reticulum (sER).
FIGURE 6-2 Two light micrographs at different magnifications of chromatolytic cell bodies from the spinal cord of a dog that had spinal nerves transected. The cell bodies are swollen, nuclei are shifted to an eccentric position, and chromatophilic substance is lost except for small amounts accumulated marginally. Nissl’s stain (left, ×200; right, ×400).

Synaptic sites on dendrites are distinguished by a band of electron-dense cytoplasm of variable thickness that lines a region of postsynaptic membrane that faces a presynaptic element across a synaptic cleft (Fig. 6-3). The dense material represents proteins (receptors, channels, enzymes, etc.) responsible for postsynaptic activity.
FIGURE 6-3 Schematic illustration of an axodendritic synapse. The terminal bulb of an axon is separated from a dendrite (D) by a synaptic cleft (SC) containing glycoprotein material. Astrocyte processes (A) border the synaptic cleft bilaterally. Within the terminal bulb, synaptic vesicles (S) are clustered around an active zone in which an electron-dense band lines the plasma membrane. As a consequence of Ca++ influx, synaptic vesicles are able to dock with the plasma membrane and release neurotransmitter molecules by exocytosis. Vesicle membrane is recovered from the plasma membrane and conveyed, as coated vesicles (C), to smooth endoplasmic reticulum (sER) for recycling. A solitary, large, densecore secretory vesicle (possibly containing a peptide or monoamine neuroactive substance) is also shown (V), along with mitochondria, microtubules, and neurofilaments. The transverse section of the dendrite features an electron-dense band (E) along the postsynaptic membrane. Numerous microtubules and neurofilaments and a few sER profiles are evident in the dendrite.

Many neurons display numerous dendritic spines (gem-mules), the postsynaptic partner in many excitatory synapses. A spine is a short expanded process attached to the dendritic branch by a narrow stalk, like a bud on a twig (Fig. 6-4). A spine apparatus, consisting of alternating membrane sacs and dense material, may be found within the spine. Spines increase dendritic surface area and act to restrict the spread of postsynaptic excitation. For a century or more, dendritic spines were considered very static structures, but within the last 5 years, research has shown that the structure of the spine is very dynamic. Time-lapse imaging shows that adult spines can change shape by as much as 30% of their length or width within a few seconds to minutes. Furthermore, high-frequency stimulation induces protrusion of new dendritic processes as well as spine bifurcation, also within minutes. These data suggest that changes in spine number or shape may contribute to synaptic plasticity, perhaps by providing a lasting structural substrate for newly encoded memories.
Axon
The axon is a relatively long, cylindrical process that originates from the axon hillock of the cell body and ends in terminal branches and synaptic boutons, which are described below. While the axon typically emerges from the cell body, in some neurons found in parasympathetic ganglia or in the brain, the axon originates as a branch of a dendrite. Except at its termination, axonal branches are sparse. When branches are present along the course of the axon, they emerge at right angles (from nodes in the case of myelinated axons) and are called collateral branches. Axoplasm, the cytoplasm of the axon, contains microtubules, neuro-filaments, mitochondria, and sER. Devoid of rER, axoplasm is not well stained by hematoxylin and eosin and other routine histologic stains.
FIGURE 6-4 A pyramidal neuron from the cerebral cortex is shown (Golgi silver impregnation). This multipolar neuron has a pyramidal cell body from which apical and basal dendrites arise. A single axon (not visible) leaves the lower surface of the cell body. The insert, an enlargement of the middle of the apical dendrite, shows dendritic spines.

The initial segment of the axon is a site just distal to the axon hillock, where action potentials normally originate. It is narrower than the rest of the axon. Ultrastructurally, bundled microtubules are present and electron-dense material is evident along the inner surface of the plasma membrane of the initial segment. The density represents protein accumulation associated with ion channels and pumps. Similar electron-dense protein accumulations are also found at nodes of myelinated axons.
A slow flow of cytoplasm (1 mm/day) and a slow transport of cytoskeletal elements (10 mm/day) progress along the axon, from axon hillock to terminal branches (anterograde transport). In addition, axons have a fast transport capability (up to 400 mm/day) involving microtubule-linked movement of mitochondria and constituents packaged in vesicles. There is also a retrograde transport mechanism that rapidly conveys remnants of organelles from terminal branches back to lysosomes within the cell body. Viruses (e.g., rabies) and neurotoxins (e.g., tetanus toxin) can also be carried to the cell body by this route.
Axons are capable of regenerative conduction; that is, they convey an excitation signal to the end of the axon with the same magnitude as it had when it began at the initial segment. Large axons conduct more rapidly than small ones, but to further increase conduction velocity, large axons are myelinated (coated with a myelin sheath formed by glial cells). Small nonmyelinated axons are ensheathed by glial cells in peripheral nerves, but they are not ensheathed at all in the CNS.
Axon degeneration may be causally linked to the documented physiologic and behavioral deficits observed in aged animals. Traditionally, the decline in memory function and behavioral dysfunction that accompany normal aging in animals have been attributed to loss of neurons in certain areas of the brain. Recent data, however, show that aging does not induce prominent cell loss in the brain, but rather leads to degeneration of axons that innervate certain forebrain structures.
Terminal branches (telodendrites)
Axons terminate by successively branching (Fig. 6-1). The collective terminal branches constitute the axon terminal (telodendritic) zone of a neuron. Each terminal branch ends in an expansion called a terminal synaptic bulb (synaptic bouton). Along the non-myelinated terminal branches, synapses occur at multiple focal swellings called axonal varicosities (preterminal boutons). The number of axonal varicosities is not insignificant; a single hippocampal cell axon makes 50,000 synapses over a distance of only 200 mm. Both axonal varicosities and terminal synaptic boutons are sites where neurotransmitter molecules are packaged and stored within synaptic vesicles.
The most common synaptic vesicle is spherical (40 to 50 nm in diameter) with an electron-lucent (agranular) core (Figs. 6-3 and 6-5). Such vesicles may contain any one of a number of neurotransmitters. Electron-lucent synaptic vesicles that appear flattened when exposed to solutions of high osmolarity are associated with inhibitory synapses. Some neurons have spherical vesicles (40 to 60 nm in diameter) with an electron-dense (granular) core. The electron-dense synaptic vesicles usually contain dopamine or nor-epinephrine molecules.
Synaptic vesicle proteins are synthesized in the cell body and transported rapidly to axon terminal sER, where they supplement local production of synaptic vesicles from recycled vesicular membrane. Transporter proteins in vesicle membrane load newly formed synaptic vesicles with neurotransmitter molecules that were also recycled. Linked by cytoskeletal actin filaments, synaptic vesicles are clustered together, in ready reserve for plasma membrane docking and exocytosis during synaptic activity (Fig. 6-3). In addition to synaptic vesicles, terminal branches may contain secretory vesicles that store neuroactive peptides (several dozen have been identified). Peptides are found in spherical, electron-dense vesicles that are relatively large (100 to 200 nm in diameter). The secretory vesicles are synthesized in the cell body and transported to preterminal and terminal synaptic boutons for storage and release. The peptides generally act as neuromodulators (agents that augment neurotransmitter effects). Secretory vesicles of certain hypothalamic neurons contain peptide hormones (e.g., vasopressin and oxytocin are stored and released in the neurohypophysis).
Sprouting of new terminal branches and degenerations of existing branches in response to environmental change is a major mechanism of neural plasticity, enabling neural development and subsequently behavioral modification and learning.
Classification
Neurons are anatomically classified as unipolar, bipolar, or multipolar according to the number of processes that emanate from the cell body (Fig. 6-6).
In a unipolar neuron, the cell body gives off a single axon that soon bifurcates into central and peripheral branches. The peripheral branch terminates in receptors that are sensitive to environmental energy. The central branch conveys the environmentally induced excitation into the CNS. Unipolar cell bodies are found in sensory ganglia located in roots of cranial and spinal nerves. Mammalian unipolar neurons are often referred to as pseudounipolar, because they originate as bipolar cells and only become unipolar during development. The unique geometry of unipolar sensory neurons can lead to the generation of spontaneous discharge in the cell bodies, potentially disrupting the fidelity of afferent signaling in normal animals. More importantly, following nerve injury, discharge originating ectopically within spinal ganglion cells is greatly augmented and can be a major contributor to neuropathic dysesthesias and chronic pain.
In a bipolar neuron, two processes emanate from the cell body, which is situated either within the axon (vestibulocochlear afferent neurons; bipolar cells of the retina) or at the juncture of the axon and a solitary dendrite (olfactory afferent neurons). Like unipolar neurons, bipolar neurons are afferent neurons that convey sensory information to the CNS.
FIGURE 6-5 Schematic illustrations of types of central nervous system synapses. 1. Axosomatic synapse with electron-lucent, spherical, synaptic vesicles. 2. Axosomatic synapse with synaptic vesicles that are flattened (an artifact associated with inhibitory synapses). 3. Axosomatic synapse with electron-dense synaptic vesicles. 4. Axodendritic synapse. 5. Axoaxonic synapse in which one terminal bulb synapses on another (associated with presynaptic inhibition). 6. Synapse on an axon hillock, close to the initial segment of the axon. 7. Axodendritic synapse on a dendritic spine. 8. Relatively elaborate synapse on a dendritic spine. 9. Dendritic spine exhibiting a spine apparatus (smooth endoplasmic reticulum plus electron-dense material). 10. Three axonal varicosities making synapses in passing (en passant).

FIGURE 6-6 Schematic illustration of a bipolar neuron (left), a unipolar neuron (center), and a multipolar neuron (right). Neurons are anatomically classified according to the number of processes emanating from the cell body. Unipolar and bipolar neurons are sensory. Most neurons are multipolar; however, their shapes vary considerably. The cytoplasm of the cell bodies features clumps of chromatophilic substance (Nissl substance).

In a multipolar neuron, the cell body gives rise to multiple branches, several dendrites, and an axon. Nearly all of the billions of neurons comprising the CNS are multipolar, as are the neurons contained in autonomic ganglia of the PNS.
Regions of a Neuron
A typical neuron becomes excited at its input region, conducts excitation to its output region, and transmits excitation via chemical secretion at synapses (Fig. 6-1). Reception, conduction, and transmission of excitation require functionally different ion channels and cellular features. Thus, an individual neuron has distinct regions: (a) an input region or dendritic zone, where excitation is initially received; (b) an output region or telodendritic (axon terminal) zone, where excitation is transmitted to other cells; (c) an axon, which conducts excitation between the dendritic and axon terminal zones; and (d) a cell body, which nurtures the cell.
In a typical multipolar neuron (Fig. 6-1), the receptive dendritic zone features a large surface area encompassing the cell body and highly branched dendrites. An elongated, cylindrical axon originates from the cell body. The axon terminal zone is a highly branched region located at the distal end of the axon. The terminal branches feature localized expansions (boutons or bulbs) where neurotransmitter molecules are stored and released at synapses.
In the case of a unipolar afferent neuron, the cell body is situated along the axon and the dendritic zone consists of receptors that change environmental energy into neural excitation. Dendritic zones of bipolar afferent neurons may involve receptors (olfaction) or synaptic contact with receptor cells (in the retina and inner ear). From dendritic zones, excitation is conveyed along the axon to terminal branches within the CNS.
The protein composition of the plasma membrane is necessarily different at each functional region of a neuron. For example, dendritic zone membrane has ligand-reactive protein receptors that open ion channels, either directly or through second messengers. Here the term “receptor” refers to membrane proteins interacting with extracellular physiologic signals and converting them into intracellular effects. Axon membrane has voltage-gated Na+ channels that enable membrane polarity reversal and regenerative conduction. The membrane of terminal branches and synaptic boutons has voltage-gated Ca++ channels (Ca++ is involved in the release of neurotransmitter molecules) and membrane-associated receptors for reuptake of released neurotransmitter.
Neuronal Communication
Neurons communicate with one another, with glial cells, and with the muscles and glands that neurons innervate. Alterations in excitation communicated from neuron to neuron (neural circuits) constitute the basis of nervous system function.
Among the billions of neurons that comprise the nervous system, the primary means of communication is localized release of neurotransmitter molecules at interneuronal chemical synapses. Chemical synaptic arrangements are also encountered between efferent neurons and the muscles and glands they innervate (see Peripheral Nervous Tissue section). A few neurons communicate through gap junctions (electrotonic synapses), especially in invertebrates and fish. In mammals, gap junction communication is common between neuroblasts during embryonic development, but it is relatively rare between mature neurons. Other neurons communicate by producing a gas that passes freely through neuro-nal membranes (e.g., the gases nitric oxide and carbon monoxide are increasingly appreciated as major neurotransmitters; NO and CO transmit signals between neurons by binding to a heme moiety at the active site of soluble guanylyl cyclase, leading to an increase in the intracellular second messenger molecule cyclic guanosine 5′-monophosphate [cGMP]).
Interneuronal Chemical Synapses
An interneuronal chemical synapse is a site of morphologic specialization where one neuron influences the excitability of another by releasing neurotransmitter molecules from synaptic vesicles. A majority of neurotransmitters are biogenic amines (e.g., glutamate, glycine, dopamine, norepinephrine, serotonin, acetylcholine, etc.). Generally, each neurotransmitter can interact with a variety of membrane receptors.
A receptor is a site on a membrane protein to which a neurotransmitter binds briefly. The receptor protein may be a channel that undergoes reconfiguration to allow passage of selective ions, or the receptor may be coupled to a G-protein cascade that opens ion channels either directly or indirectly by activating second messengers (such as cyclic adenosine monophosphate [cAMP]). Second messengers provide a means of amplifying the scope and impact of a neurotransmitter signal as well as prolonging its time course. Autoreceptors (receptors in presynaptic plasma membrane that influence neurotransmitter synthesis and release) and neuro-modulators (peptides released from secretory vesicles) typically act via second messengers.
Ionotropic membrane receptors represent one large class of neurotransmitter receptors in which the receptor protein is part of an ion channel–receptor protein complex. When activated, the complex opens or closes the ion channel, resulting in a change in membrane permeability. Metabotropic receptors represent the other large class of neurotransmitter receptors in which the receptor protein acts via another protein such as a G protein to activate or inhibit enzymes and possibly open, close, or modify ion channels, ultimately leading to a change in cell function.
Based on the ion channel or the G protein to which a receptor is coupled, the receptor may be excitatory or inhibitory. Thus, it is the nature of the receptor type, rather than the neurotransmitter itself, that determines the function of a synapse (e.g., excitatory or inhibitory, short or long acting, etc.). Nonetheless, some neurotransmitters (e.g., glutamate) are associated with excitatory synapses, and others (e.g., glycine) are associated with inhibitory synapses. It is worth noting that glutamate receptors are the most prevalent excitatory neurotransmitter receptors in the brain, and they mediate almost all excitatory communications between CNS neurons. Thus, it is perhaps not surprising that epileptic seizures are a problem of overactivation of brain neurons that are driven mainly by glutamate synapses.
Most synapses between neurons involve terminal synaptic boutons of one neuron contacting the input region of another neuron, forming axodendritic or axosomatic synapses (Fig. 6-5). However, among the trillions of synapses in the nervous system, every synaptic combination has been observed, including axo-axonic, dendrodendritic, dendrosomatic, somatodendritic, and somatosomatic synapses.
The input region of a typical multipolar neuron receives thousands of synaptic contacts. Excitatory and inhibitory synaptic effects are collectively summated and integrated at the cell body of the target neuron, so that the cell body membrane potential continually registers the net effect of total synaptic input to the neuron. In turn, the cell body affects the membrane potential at the nearby initial segment of the axon. At any moment, the collective synaptic input to a neuron will be sufficient to trigger an action potential at the initial segment of the axon or be insufficient to do so.
The influence that one neuron has on another depends on the number of synaptic contacts it makes with the target neuron and where the synapses are positioned. Synapses close to the initial segment will have much greater influence triggering an action potential than will synapses on distal dendrites.
Synaptic Ultrastructure and Function
Ultrastructurally, an interneuronal chemical synapse may be identified by the juxtaposition of a presynaptic element, a synaptic cleft, and a postsynaptic membrane (Fig. 6-3). The presynaptic element contains multiple synaptic vesicles clustered around an active zone indicated by electron density (protein accumulation) just inside the plasma membrane. The synaptic cleft (in the CNS, approximately the same width as the general intercellular space, 20 to 30 nm wide) contains a protein that holds presynaptic and postsynaptic membranes together. The postsynaptic somatodendritic membrane contains many types of protein receptors that accumulate to form functional microdomains opposite pre-synaptic terminal boutons that release neurotransmitters. These domains are associated with postsynaptic densities that, under the electron microscope, appear as electron-dense material facing the presynaptic active zone. The postsynaptic density, which consists of adhesive proteins, such as cadherin–catenin complexes, receptor proteins, subsynaptic scaffolding proteins, and associated components of the cell’s cytoskeleton, is involved in the stabilization and trafficking of receptors and in signal transduction.
When an action potential arrives at the end of an axon, it passively depolarizes the presynaptic element (e.g., terminal synaptic bouton). Voltage-sensitive channels in the presynaptic membrane open to allow Ca++ influx. Elevated cytoplasmic Ca++ activates enzymes that phosphorylate synaptic vesicle proteins responsible for reversibly linking vesicles to cytoskeletal actin filaments and merging vesicles with plasma membrane. This mobilizes synaptic vesicles, enabling them to dock with the plasma membrane and release several thousand neurotransmitter molecules by exocytosis. The molecules diffuse into the synaptic cleft and bind with various receptors and transporters.
Synapses are very dynamic structures and there is constant movement of receptors into and out of synaptic membranes. Recent data have shown that rapid gain and loss of neurotransmitter receptors from synaptic sites are accounted for by endocytosis and exocytosis, as well as by lateral diffusion in the plane of the membrane. These events are interdependent and are regulated by neuronal activity and interactions with scaffolding proteins. Thus, it is known that activity-dependent elevation in postsynaptic intracellular calcium concentration triggers rapid receptor immobilization and local accumulation on the neuronal surface. Neurotransmitter receptor movement into and out of the synaptic membrane is one of the core mechanisms for rapidly changing the number of functional receptors during synaptic plasticity.
Synaptic activity ceases when neurotransmitter molecules are removed from the synaptic cleft (or degraded in the case of acetylcholine). Molecules are actively transported intracellularly by transporters (protein pumps) located in the presynaptic membrane or in membranes of adjacent glial cells. Thus, neurotransmitter molecules are recycled to minimize the need for synthesis within presynaptic cytoplasm. As well, synaptic vesicle membrane is recycled. Vesicle membrane is extracted from the presynaptic plasma membrane and transported as coated vesicles to sER within the presynaptic cytoplasm (Fig. 6-3).
NEUROGLIA
Neuroglia (gliocytes) comprise over 90% of the cells that make up the nervous system. Recent studies have shown that glia are not simply the support structure in which neurons are embedded. While the biology of glial cells is poorly understood, it is clear that glial–neuronal and glial–glial interactions are essential for many of the critical functions that occur in the nervous system. Neurons communicate with glial cells by releasing ATP at synapses and from axons during conduction. Glial cells have vital roles in neuronal development, activity, plasticity, and recovery from injury.
Neuroglia are endowed with a rich assortment of ionic channels, neurotransmitter receptors, and transport mechanisms that enable the gliocytes to respond to many of the same signals acting on neurons and also to modulate the neuronal response. In parallel to the serial flow of information along chains of neurons, glia communicate with other glial cells through intracellular waves of calcium and via intercellular diffusion of chemical messengers. By releasing neurotransmitters and other extracellular signaling molecules, glia can affect neuronal excitability and synaptic transmission and perhaps coordinate activity across networks of neurons. Moreover, glia secrete cytokines, growth factors, and other trophic factors that dictate long- and short-term survival of neurons as well as the elaboration and retraction of synaptic connections.
From a histologic perspective, neuroglial cells are relatively small. With routine histologic stains, only their nuclei and cell bodies are evident, and thus in tissue sections they are distinguished primarily by the size and shape of their cell bodies and the size and chromatin pattern of their nuclei. However, the use of modern immunocytochemical staining for specific cell surface or internal markers allows definitive identification of glial cells in brain sections. It should be noted that unlike mature neurons, glial cells remain capable of mitosis, and thus they can give rise to tumors of the nervous system.
Except for microglial cells, which migrate into the CNS from mesoderm, CNS gliocytes are derived from the ectodermal cells that form the embryonic neural tube. Neurolemmocytes of the PNS are derived from the embryonic neural crest (as are neurons that have cell bodies in ganglia).
Central Nervous System Gliocytes
The gliocytes of the CNS include astrocytes, oligodendrocytes, microglia, and ependymal cells.
Astrocytes
With routine stains, astrocytes are identified by their pale, ovoid nuclei, which are largest among glial nuclei. With silver stains, astrocytes exhibit numerous processes that contain glial fibrils. In white matter, the processes are long, slender, and moderately branched; in gray matter, the processes appear shorter and highly branched. Thus, white matter is said to contain fibrous astrocytes, whereas gray matter contains protoplasmic astrocytes (Fig. 6-7).
Under the electron microscope, astrocytes feature packed bundles of intermediate filaments (8 nm in diameter) and pale cytoplasm. The glial filaments, which are composed of glial fibrillar acidic protein (GFAP), are unique to astrocytes and form the glial fibrils seen with light microscopy. The filaments are denser in fibrous astrocytes than in protoplasmic ones.
Adjacent astrocytes are joined by gap junctions. In response to local stimulation, a widening excitatory wave of elevated cytoplasmic Ca++ can spread outward from cell to cell via the gap junctions. The adjacent astrocytes are also linked by small, buttonlike adhering junctions (spot desmosomes).
Astrocyte processes terminate in expansions called end feet. Collections of end feet form a glial-limiting membrane to which pia mater is attached at the CNS surface. End feet are prominent in the subependymal glial layer, and they form septa in the spinal cord. End feet cover vessels within the brain and spinal cord, and they are believed to be responsible for inducing formation of tight junctions between capillary endothelial cells (a basis for the blood-brain barrier further described on page 115).
Astrocytes provide structural support through their glial fibrils, but in addition, these cells play a critical role in several aspects of brain function. By storing glycogen and releasing glucose, they represent a source of reserve energy. Astrocyte plasma membranes have ionic pumps that regulate K+ throughout the narrow extracellular space of the CNS. Astrocyte processes insulate synapses and release substances that modulate synaptic sensitivity. Astrocytes can also take up neurotransmitter molecules from the synaptic cleft to stop ongoing synaptic activity.
Recent data have revealed that astrocyte activity is probably determined by neuronal activity and that astrocytes have the capacity to signal not only to each other, but also back to the neurons. Furthermore, since astrocytes can sense synaptic activity, it is thought that they are pivotal intermediaries between neurons and the brain microcirculation. In this regard, increased synaptic activity triggers calcium waves in astrocytes, causing their end feet to release substances that cause local vasodilation. Thus, astrocytes can act to couple brain activity not only to energy demands but also to blood flow requirements.
Finally, astrocytes seem to have an immune function; they can present antigens to T lymphocytes and can secrete a wide array of chemokines and cytokines, allowing them to influence T helper cell response and monocyte/microglia effector functions. It should be noted that the reaction of astrocytes following brain trauma or in certain pathologic states is characterized by astrocyte proliferation and hypertrophy, elongation of astro-cyte processes, up-regulation of various intermediate filaments, and, in cases of severe trauma or pathology, the formation of a dense glial scar. This process is referred to as astrogliosis and the glial scar is formed by the hypertrophied processes of reactive astrocytes filling in the space that results from loss of myelin and neurons.
FIGURE 6-7 Central nervous system gliocytes are drawn as they appeared following Golgi silver impregnation of monkey cerebral cortex (×700). The insert at the bottom shows an astrocyte nucleus (astr) and an oligodendrocyte nucleus (oligo) as they appear in white matter stained with Luxol blue and hematoxylin. An axon (a) surrounded by a myelin vacuole and neurokeratin can be seen. (Adapted from Weiss L. Cell and Tissue Biology. A Textbook of Histology. 6th Ed. Baltimore: Urban & Schwarzenberg, Inc., 1988.)

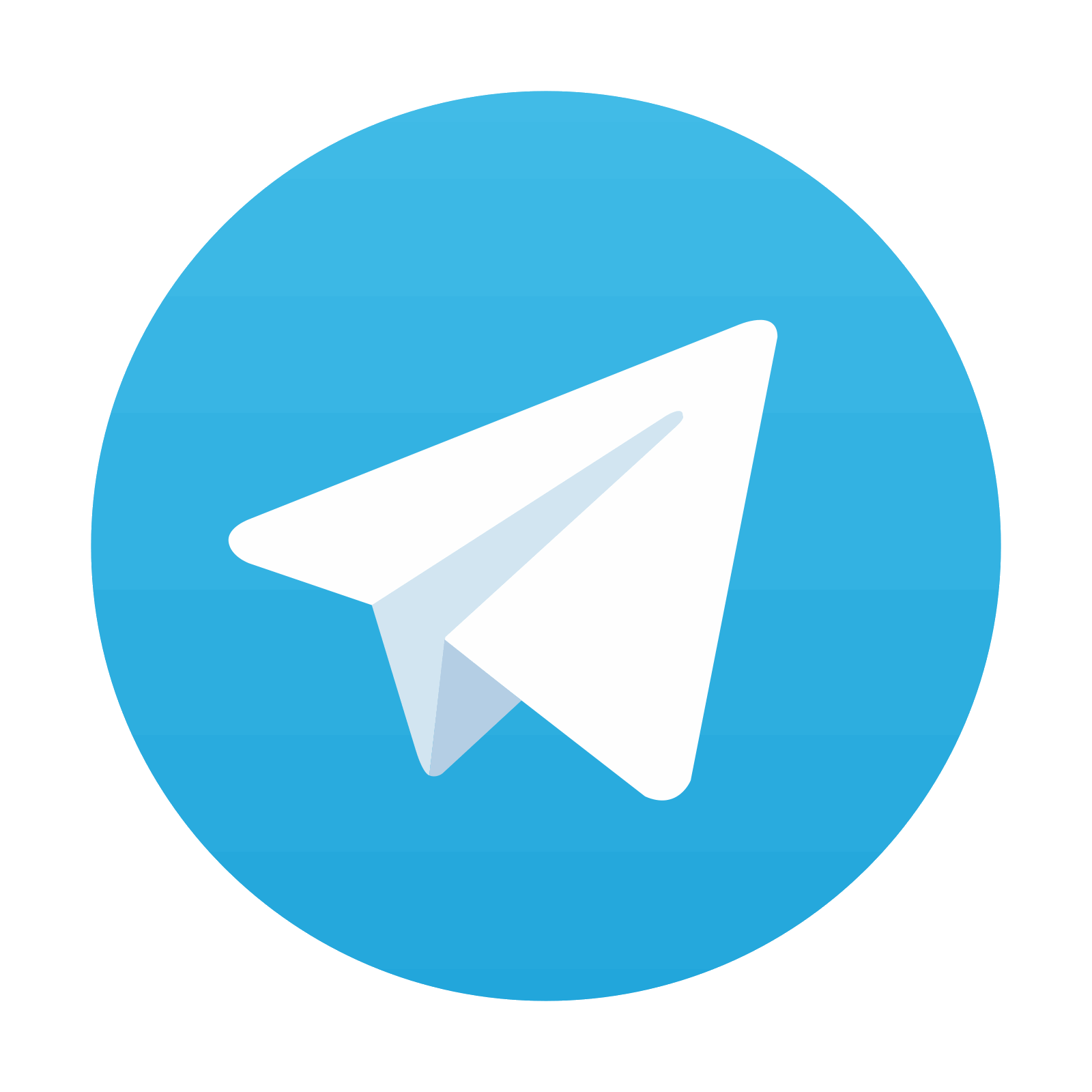
Stay updated, free articles. Join our Telegram channel
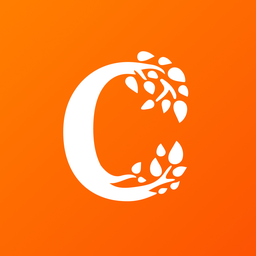
Full access? Get Clinical Tree
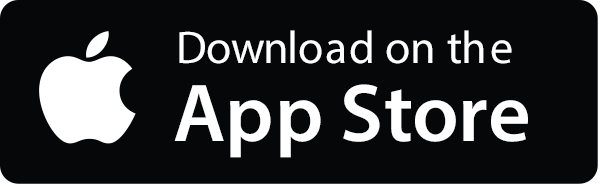
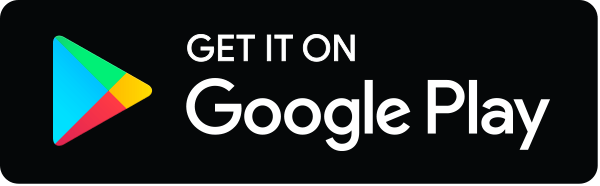