Chapter 17 In mammals, a series of paired mesodermal structures referred to as somites develop caudal to the otic placodes. These bilateral structures, which are derived from paraxial mesoderm, are located lateral to the developing neural tube and notochord. Somites, transient structures which are formed in a cranio‐caudal sequence, are essential for the segmental arrangement of the spinal column and associated spinal nerves. In the canine embryo during the third week of gestation, the outlines of somites first become visible. The number of somite pairs, which is constant for a given species, is usually one pair per vertebra. Differentiation of somites commences around the fourth week of gestation. By the fifth week of gestation, when somite formation is complete, those formed at an earlier stage have already undergone further differentiation. Initially, cells at the periphery of a somite have the appearance of epithelial cells, while those that are centrally located are not arranged in a defined pattern. With the commencement of differentiation, the epithelial‐like cells of the medial and ventral walls of each somite differentiate into mesenchymal cells. These differentiating areas are referred to as sclerotomes and the cells from these regions give rise to connective tissue, including cartilage and bone (Fig 17.1). The epithelial‐like cells of the dorsal and lateral walls of each somite form structures referred to as dermomyotomes. Cells from the dorso‐medial and dorso‐lateral borders of the dermomyotome form a distinct layer, the myotome, which gives rise to skeletal muscle; cells of the central region of the dermomyotome give rise to the dermatome which contributes to the formation of the dermis of the skin. Figure 17.1 Cross‐sections through embryos at different stages of development showing structures which are derived from somites. A. Location of somites early in gestation. B. Formation of sclerotomes and dermomyotomes from somites. C. Separation of dermatome from myotome and formation of vertebral primordium. D. Division of myotomes into dorsal epimeres and ventral hypomeres. At this stage, innervation of both muscle masses by branches of spinal nerves occurs. E. Section through abdominal region showing the location of epaxial muscles which develop from epimeres and hypaxial muscles which are derived from hypomeres. Differentiation of somites is influenced by factors produced by adjacent structures, including the notochord, neural tube, lateral plate mesoderm and surface ectoderm. Sonic hedgehog, produced by the notochord and the floor plate of the neural tube, induces the ventro‐medial region of the somite to become the sclerotome. The sclerotome expresses the factors paired box‐1 (Pax‐1) and Pax‐9, which induce the cells of the sclerotome to undergo mitosis. The sclerotomal cells lose their intercellular adhesion molecules and undergo transformation into mesenchymal cells which migrate towards the notochord and neural tube (Fig 17.1). Skeletal, cardiac and smooth muscle, which develop from mesoderm, comprise the musculature of the body. Skeletal musculature is derived from paraxial mesoderm which, in the cranial region, forms somitomeres and, in the regions caudal to the otic placodes, forms somites. Splanchnic mesoderm gives rise to cardiac muscle and also to the smooth musculature of the digestive and respiratory tracts. Smooth muscle of blood vessels and the arrector pili muscles are derived from mesenchyme in the regions where these structures develop. The myotome forms the skeletal muscles of the trunk, neck and limbs. In response to two factors secreted by the neural tube, neurotrophin 3 and Mat‐1, the dermatome differentiates and gives rise to cells which contribute to the formation of the dermis of the body with the exception of the cranial region. The major contribution to the formation of the dermis, however, is from somatic mesoderm of the body wall. Under the influence of Wnt proteins produced by cells in the dorsal wall of the neural tube, the dorso‐medial cells of the dermomyotome become activated, forming the medial myotomal segment of the dermomyotome which expresses the muscle‐specific gene product Myf‐5. The action of Wnt proteins from the body wall and Bmp from the lateral plate mesoderm on the dermomyotome leads to formation of the lateral myotomal segment of the dermomyotome and promotes MyoD expression. The transcription factor MyoD influences muscle differentiation. Under the influence of the neurotrophin Nt‐3, expressed by cells in the dorsal region of the neural tube, cells of the central region of the dermomyotome are induced to differentiate and contribute to the formation of the dermis. Cells of the myotome proliferate and give rise to progenitor muscle cells, myoblasts. The myoblasts derived from the dorso‐medial region of the myotome form a structure referred to as the epimere, while a grouping of myoblasts from the dorso‐lateral region of the myotome form the hypomere (Fig 17.1D). The epimere is located dorsal to the transverse processes of the developing vertebrae, while the hypomere is located ventral to the transverse processes. Spinal nerves develop in association with each developing somite and each nerve gives off a dorsal branch to an epimere and a ventral branch to a hypomere. As epimeres and hypomeres are somite‐derived, the muscle groupings formed are initially arranged segmentally along a cranial–caudal axis. Subsequently, the segmentally‐arranged epimeric muscles fuse, forming the extensor muscles of the vertebral column, comprising the transverso‐spinalis system, the longissimus system and the ileo‐costalis system. Collectively, the muscles of these systems are referred to as the epaxial musculature. Epaxial myoblasts are induced to proliferate by the factors Wnt‐1 and Wnt‐3a, which are produced by cells in the dorsal region of the neural tube together with low levels of Shh, which arise from cells located in the ventral region of the neural tube. The segmentally‐arranged hypomeric muscle bundles proliferate and extend ventrally into the somatopleure of the body wall, forming the primordial musculature of the body wall which initially remains segmented. Subsequently, with the exception of the thoracic region, the hypomeres fuse. In the cervical region, the fused hypomeres give rise to the ventral musculature of the neck. The hypomeres in the thoracic region, while retaining their segmented arrangement, give rise to three muscle layers, the external and internal intercostal muscles and the transverse thoracic muscles. Ribs develop in the undifferentiated mesenchyme between the segmentally‐arranged intercostal muscles. In the abdominal region, individual hypomeres fuse forming a continuous muscular sheet, which subsequently gives rise to three muscle layers, the external and internal oblique abdominal muscles and the transverse abdominal muscles (Fig 17.1E). The ventral portions of the proliferating hypomeres which separate from the main muscle bands fuse, forming the primordium of the rectus abdominis muscle. Myoblasts from the hypomeres in the lumbo‐sacral region give rise to the sublumbar muscles, the psoas major and psoas minor muscles and the quadratus lumborum muscles. In the sacro‐caudal region, myoblasts give rise to the muscles of the pelvic diaphragm, comprised of the coccygeus and the levator ani muscles. Muscles derived from hypomeres are collectively referred to as hypaxial muscles. Hypaxial myoblasts originating from the dorso‐ventral edges of the somites are probably specified by Wnt and Bmp‐4 proteins expressed by the lateral plate mesoderm. The musculature of the limbs develops from myoblasts which migrate from hypaxial musculature to the limb buds. The skeletal musculature of the head orginates from myoblasts which arise from somitomeres and migrate to the pharyngeal arches. Musculature of the head is discussed in Chapter 22. Most of the smooth muscle fibres of the body differentiate from cells which develop from splanchnic mesoderm. The origin of smooth muscle fibres of blood vessels is generally considered to be mesenchyme, while the ciliary and pupillary sphincter muscles of the eye are derivatives of neural crest cells. Cells derived from splanchnic mesoderm surrounding the cardiac tube give rise to cardiac muscle. Unlike skeletal muscle fibres which are formed by the fusion of individual myoblasts, cardiac muscle fibres are formed by the growth and differentiation of single cardiac myoblasts. Growth of cardiac muscle occurs by the formation of new myofilaments. End‐to‐end adhesion of adjacent cardiac muscle cells occurs at specialised intercellular junctional complexes called intercalated discs. When cardiac muscle cells adhere to each other in a linear fashion, such a structure is referred to as a cardiac muscle fibre. During cardiac development, a group of myoblasts differentiate into special cells which form Purkinje fibres. These cells increase in size, undergo a reduction in the myofibrillar content and acquire an increased concentration of glycogen in their cytoplasm. Purkinje fibres form the intrinsic conducting system of the heart. Under the influence of the myogenic transcription factors Wnt, Shh, MyoD and Myf‐5, cells derived from myotomes are induced to form myoblasts. The myoblasts initially undergo a period of mitosis triggered by fibroblast growth factors and transforming growth factors. As the concentration of these growth factors decreases, myoblasts cease to divide and begin to elongate. The spindle‐shaped myoblasts fuse end to end and disintegration of their cell membranes at their points of contact results in the formation of long, multinucleated syncytia, termed myotubules. The remaining portions of the cell membranes of adjacent myoblasts, which do not break down, form a continuous external lamina referred to as the sarcolemma. Fusion requires specific molecules, including cadherins, which promote the cell‐to‐cell adhesion of the developing myoblasts. The final stages in skeletal myotubule differentiation involve the production of specific myofilaments composed of the contractile proteins, actin, myosin, tropomyosin and troponin, in a repeating pattern along the length of the myotubule. Myofilaments of actin and myosin become arranged into contractile units referred to as sarcomeres. When arranged in a linear fashion, sarcomeres form myofibrils. Collections of myofibrils grouped together in parallel formation constitute a skeletal muscle fibre. Nuclei are arranged along the periphery of the fibre and mitochondria become orientated parallel to the long axis of the sarcomeres. A thin layer of connective tissue which surrounds individual skeletal muscle fibres is referred to as the endomysium. Bundles of muscle fibres, referred to as fascicles, are surrounded by a connective tissue layer, the perimysium. The fibrous sheath, composed of dense connective tissue surrounding an entire skeletal muscle, is referred to as the epimysium. Undifferentiated myoblasts, located between the sarcolemma and the basal lamina of the muscle, are referred to as satellite cells. During postnatal life, satellite cells or their progeny can fuse with existing muscle fibres thereby increasing fibre length. Post‐mitotic fusion of myoblasts involves adhesion molecules, including N‐CAM and V‐CAM, cadherins and integrins. Muscle damage is repaired by division and subsequent fusion of satellite cells. Innervation is an essential requirement for normal muscle development. Muscle fibres are first innervated by motor nerve fibres and later by sensory nerve fibres, the latter inducing formation of the specialised stretch receptors of muscle, the intrafusal muscle fibres. The skeletal system, which consists primarily of bone and cartilage, provides a supporting framework for other body structures and also protects internal organs. The majority of skeletal structures are composed of bone, while cartilage is associated with bone on articular surfaces, at growth plates and at interosseous connections in addition to forming the embryonic skeleton. Cartilage also functions as a supporting tissue in structures such as the larynx, trachea and external ear. The cells which give rise to the skeletal system, excluding skeletal structures of the head, develop from paraxial and lateral plate mesoderm. The skeletal structures of the head arise from mesenchymal cells which are of neural crest origin. The primordial cells of cartilage, chondroblasts, are of mesenchymal origin. The commitment of mesenchymal cells to form cartilage is triggered by transcription factors Pax 1 and Scleraxis, which activate cartilage‐specific genes. At the particular sites where cartilage formation occurs, mesenchymal cells form aggregations and differentiate into chondroblasts. Cells committed to this chondrogenic fate switch their expression of type IIA collagen to the expression of cadherin 2 (also known as neural cadherin), neural cell adhesion molecule 1, tenascin‐C and the transcription factor Sox‐9. These molecules mediate cell‐to‐cell interactions and are essential for maintaining the expression of Sox‐9, which is one of the earliest acting transcription factors in the chondrogenic programme. As the presumptive chondrogenic cells develop into chrondoblasts, the hyaluronan content of the extracellular matrix undergoes a significant reduction. The continued differentiation of the chondroblasts is promoted by Bmp factors, which maintain the expression of Sox transcription factors (Sox‐9, 5 and 6) by chondroblasts, which in turn augments the expression of collagen types II, IX, and XI and the major proteoglycan of cartilage, aggregan. As differentiation continues, chondroblasts lose their cytoplasmic processes, become rounded and produce the extracellular matrix of cartilage which is composed of glycosaminoglycans, proteoglycans and collagen fibres (Fig 17.2). Based on the types and distribution of fibres in their matrices, three types of cartilage are recognised: hyaline, elastic and fibrocartilage. Hyaline cartilage contains type II collagen fibres, while elastic cartilage contains type II collagen fibres with elastic fibres scattered throughout the matrix. Fibrocartilage contains dense, coarse type I collagen fibres arranged in parallel bundles throughout the matrix. This distribution of collagen fibres accounts for the high tensile strength of fibrocartilage. Figure 17.2 Stages in the formation of cartilage from mesenchymal cells (A to D). When they become entrapped in the matrix which they produce, chondroblasts are referred to as chondrocytes. The spaces occupied by cells in cartilage are known as lacunae. The mesenchymal cells surrounding the developing cartilaginous mass give rise to fibroblasts which form a connective tissue sheath, the perichondrium, consisting of an outer fibrous layer and an inner chondrogenic layer. There is a positive reciprocal interaction between chondrocytes and the cells of the perichondrium. Indian Hedgehog secreted from pre‐hypertrophic and hypertrophic chondrocytes promotes the maturation of cartilage and perichondrium. In the perichondrium, parathyroid‐related peptide, PTHrP, is up‐regulated in response to Hedgehog signalling and acts on prehypertrophic chondrocytes, inhibiting hypertrophy. By acting as a signal relay between Indian Hedgehog and PTHrP, Tgf‐β has a role in regulating hypertrophy of cartilage. Chondrocyte hypertrophy is accompanied by the mineralisation of the extracellular matrix (ECM) and by the production of collagen type X and matrix metaloproteinase (MMP‐13) which modifies the ECM to facilitate vascular invasion. As cartilage is avascular, chondrocytes receive their supply of nutrients and oxygen by diffusion from blood vessels in the perichondrium. If the matrix becomes calcified, thereby inhibiting diffusion, the chondrocytes die, although some can differentiate into osteoblasts. A distinguishing feature of cartilage is its ability to grow by two processes. In one of these processes, referred to as interstitial growth, chondrocytes trapped within lacunae retain their ability to divide. An individual chondrocyte can give rise to an isogenous group of up to eight cells. These new cells produce matrix components, thereby forming additional cartilage within the existing cartilaginous mass. As individual cells of an isogenous group lay down additional matrix, they become separated from each other and each cell becomes enclosed within its own lacuna. In the second growth process, referred to as appositional growth, chondrogenic cells of the perichondrium give rise to chondroblasts which deposit a new layer of cartilage on the surface of existing cartilage. Bone is a specialised connective tissue which is composed of cells, an organic matrix and a mineralised inorganic matrix. Three cell types, osteoblasts, osteocytes and osteoclasts, are associated with bone. The organic matrix, which consists of type I collagen and amorphous ground substance containing proteoglycans, accounts for approximately one‐third of the bone mass. The mineralised matrix, which makes up two‐thirds of bone mass, is composed of calcium phosphate in the form of hydroxyapatite crystals. Bone has a remarkable range of physical properties. It is relatively lightweight yet exhibits high tensile strength while retaining a degree of flexibility. It provides the supporting framework of the body, affords protection for vital structures and acts as a storehouse for inorganic minerals. Despite its strength and rigidity, bone is a constantly changing, living tissue which undergoes continual replacement and remodelling. Its structure, shape and composition may be influenced by stress forces and regional immobilisation and also by metabolic, nutritional and endocrine factors. The cells from which osteogenic cells are derived, osteoprogenitor cells, differentiate from mesenchymal cells. These progenitor cells, which have pale‐staining, oval or elongated nuclei with acidophilic to faintly basophilic cytoplasm, are the stem or reserve cells of bone. When activated, these progenitor cells differentiate into osteoblasts. In both developing and mature bone, osteogenic cells are found on or close to the internal and external surfaces of bone. The cells which are responsible for the synthesis of the bone matrix, osteoblasts, are found on the surface of developing bone. During active osteogenesis, osteoblasts are cuboidal or columnar cells with slender cytoplasmic processes which form gap junctions with adjacent osteoblasts. These cells have large round nuclei with prominent nucleoli and numerous mitochondria. Each osteoblast has a prominent Golgi apparatus surrounded by many vesicles. This histological feature is consistent with their capacity to synthesise large quanties of extracellular proteins. The extensive endoplasmic reticulum is responsible for the basophilia of their cytoplasm. Newly formed organic matrix synthesised by osteoblasts, which has not yet become calcified, is known as osteoid. When osteoid matrix becomes fully calcified, the resulting tissue is bone. Osteoblasts contribute to the process of calcification through the secretion into the matrix of small vesicles which are rich in alkaline phosphatase. Secretion occurs only during the period when these cells are producing bone matrix. Osteoblasts entrapped in the bone matrix are referred to as osteocytes (Fig 17.3). The differentiation of cells of the osteoblast lineage is divided into three stages, mesenchymal progenitors, preosteoblasts and finally osteoblasts. The transcription factor Sox‐9 is expressed in all osteoblast progenitors, while Runx‐2 is expressed at more advanced stages of differentation. Another transcription factor OSX, vital for osteoblast differentiation, is required downstream of Runx‐2. Both of these transcription factors are regulated by the major developmental signalling pathways, including Hedgehog, Notch, Wnt, Bmp and Fgf. Figure 17.3 Sequential stages in intramembranous ossification leading to the formation of a flat bone (A to D). Approximately 10% of osteoblasts become enclosed in the developing bone matrix, becoming osteoctyes. The decline in basophilic staining of osteocytes, due to the reduction in their endoplasmic reticulum content, coincides with the cessation of organic matrix production. As osteocytes become more deeply embedded in mineralised bone matrix, their cytoplasmic content decreases. The cell bodies of osteocytes reside in lacunae within the calcified matrix of bone. Their processes, which are located within channels known as canaliculi, establish contact with processes of other osteocytes and are connected at their ends by gap junctions. This contact allows for the intercellular transfer of ions and other low molecular weight molecules. In contrast to cartilage, which grows by both appositional and interstitial growth, bone increases in size only by appositional growth.
Muscular and skeletal systems
Differentiation of somites
Muscular system
Skeletal muscle
Cytodifferentiation of muscle
Smooth muscle
Cardiac muscle
Histogenesis of skeletal muscle fibres
Skeletal system
Histogenesis of cartilage
Bone formation
Cells of bone
Osteoprogenitor cells
Osteoblasts
Osteocytes
Osteoclasts
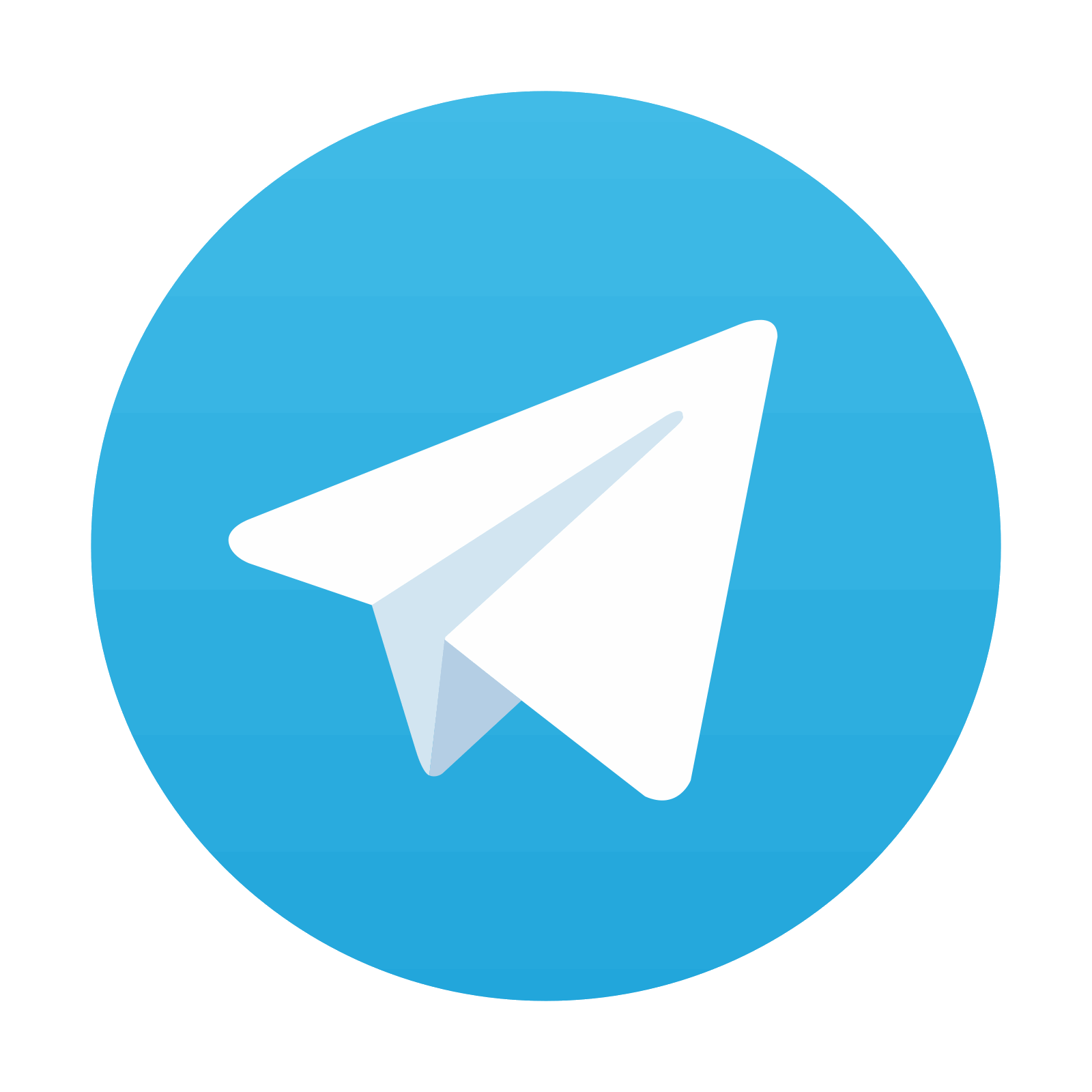
Stay updated, free articles. Join our Telegram channel
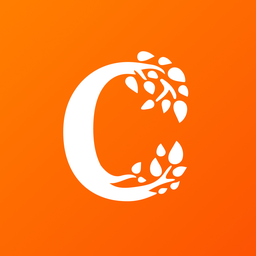
Full access? Get Clinical Tree
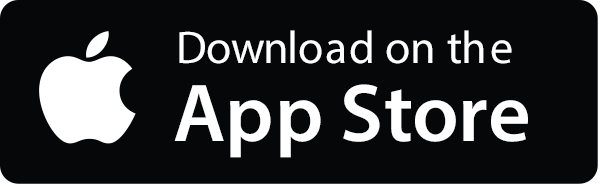
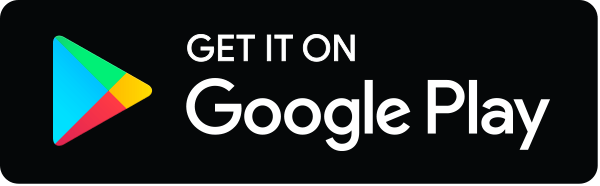